
The impulse travels along the nerve membrane as an action potential. This is entirely mediated by the receptors within the membrane. Indeed, removal of the axoplasm from the nerve fiber does not alter conduction of impulses. Nerve fibers derive their nutrition from the cell body. Interruption of a nerve fiber causes the peripheral portion to degenerate (Wallerian degeneration). The axon of a peripheral neuron is able to regenerate, as does the myelin sheath. Regeneration is the exception in most of the brain and spinal cord. Extensive research is underway to better understand the conditions that are required for central neuron regeneration to improve recovery from central neuronal injury.
Classification of Afferent Nerve Fibers
Nerve fibers are called afferent if they transmit impulses from peripheral receptors to the central nervous system (CNS) and efferent if they transmit impulses from the CNS to the periphery. Afferent nerve fibers are classified as A, B, and C on the basis of fiber diameter and velocity of conduction of nerve impulses (Table 3-1). Conduction speed increases with nerve diameter, because the larger diameter nerves have decreased longitudinal resistance to ion flux.2 The largest, and hence fastest, nerves are designated type A. Type A fibers are subdivided into α, β, γ, and δ. Type A-α1 fibers innervate muscle spindles and A-α1b innervate the Golgi tendon organ. Both A-α afferents are important to muscle reflexes and control of muscle tone.

All cutaneous mechanoreceptors (Meissner’s corpuscles, hair receptors, Pacinian corpuscles) transmit signals in type A-β fibers. Touch and fast pain are transmitted by lightly myelinated type A-δ fibers with free nerve endings. Type C fibers transmit slow pain, pruritus, and temperature sensation.
Myelin that surrounds type A and B nerve fibers acts as an insulator that prevents flow of ions across nerve membranes. Type C fibers are unmyelinated. The myelin sheath is interrupted approximately every 1 to 2 mm by the nodes of Ranvier (see Fig. 3-1).3 Ions can flow freely between nerve fibers and extracellular fluid at the nodes of Ranvier. Action potentials are conducted from node to node by the myelinated nerve rather than continuously along the entire fiber as occurs in unmyelinated nerve fibers. This successive excitation of nodes of Ranvier by an action potential that jumps between successive nodes is termed saltatory conduction (Fig. 3-3).3 Saltatory conduction allows for a 10-fold increase in the velocity of nerve transmission.2 It also conserves the membrane potential because only the membrane at the node of Ranvier depolarizes, resulting in less ion transfer than would otherwise occur. Furthermore, because depolarization is limited to the nodes of Ranvier, little energy is needed for to reestablish the transmembrane sodium and potassium ion concentration gradients necessary for signal transmission. The energy savings is more than a hundred fold. As brilliantly understated by Hartline and Colman,2 “For a nervous system such as ours, which already accounts for 20% of the body’s resting metabolic energy budget, this is not an inconsequential advantage.” If myelin did not exist, you would not be reading about it.

Evaluation of Peripheral Nerve Function
Peripheral nerves may be injured by ischemia of the intraneural vasa nervorum, as might be caused by excessive stretch of the nerve or external compression. Nerve conduction studies are useful in the localization and assessment of peripheral nerve dysfunction. Focal demyelination of nerve fibers causes slowing of conduction and decreased amplitudes of compound muscle and sensory action potentials. The presence of denervation potentials in skeletal muscle indicates axonal or anterior horn cell damage. Changes in motor unit potentials also arise from reinnervation of skeletal muscle fibers by surviving axons. Signs of denervation on the electromyogram after acute nerve injury require 18 to 21 days to develop.4 Electromyographic testing is helpful in determining the etiology of neurologic dysfunction that may occur after surgery.
The Action Potential
Electrical potentials exist across nearly all cell membranes, reflecting principally the difference in transmembrane concentrations of sodium and potassium ions. This unequal distribution of ions is created and maintained by the membrane-bound enzyme sodium-potassium ATPase, sometimes called the sodium-potassium pump. The sodium-potassium pump transfers three sodium ions out of the cell in exchange for two potassium ions brought into the cell. This causes a net transfer of positive charges out of the cell. The resulting voltage difference across the cell membrane is called the resting membrane potential. The cytoplasm is electrically negative (typically −60 to −80 mV) relative to the extracellular fluid (Fig. 3-4).5

When channels open to specific ions, the ions generally flow in the direction of their concentration gradients. An action potential is the rapid change in transmembrane potential due to the opening of sodium channels (depolarization) and rapid influx of sodium ions down the concentration gradient, reversing the net negative charge within the cell. The membrane resting potential is restored by the closing of the sodium channels and the opening of potassium channels (repolarization) after the action potential has passed. The outward flux of potassium ions down their concentration gradient restores the net negative charge within the cell. This is discussed in greater detail under the “Ion Channels” section.
Propagation of Action Potentials
Propagation of action potentials along the entire length of a nerve axon is the basis of rapid signal transmission along nerve cells. The size and shape of the action potential varies among excitable tissues (see Fig. 3-4).5
Action potentials are conducted along nerve or muscle fibers by local current flow that produces depolarization of adjacent areas of the cell membrane (Fig. 3-5). These propagated action potentials travel in both directions along the entire extent of the fiber. The transmission of the depolarization process along nerve or muscle fibers is called a nerve or muscle impulse. The entire action potential usually occurs in less than 1 millisecond.

During much of the action potential, the cell membrane is completely refractory to further stimulation. This is termed the absolute refractory period and is due to the presence of a large fraction of inactivated sodium ion channels. During the last portion of the action potential, a stronger than normal stimulus can evoke a second action potential. This “relative refractory period” reflects the need to activate a critical number of sodium ion channels to trigger an action potential.
The action potential is dynamic, which is difficult to illustrate with a static textbook image. We encourage the motivated reader to search for the text “action potential animation” on the Internet. There are many high-quality animations of the action potential that dynamically display how it propagates.
Ion Channel Evaluation
Current flowing through individual ion channels or voltage changes in a membrane can be measured with patch-clamping, a method used in electrophysiology.6 In patch clamping, an electrode is connected with a cell (or piece of membrane) with a tight seal. This electrode is able to control either the voltage or the current so that the other can be measured. Currents carried through different types of channels can be isolated by the use of specific inhibitors. For example, tetraethylammonium blocks many types of potassium ion channels, whereas tetrodotoxin blocks many types of sodium ion channels. Channels that are not normally expressed in a cell can be added through heterologous expression. With these methods, the impact of specific naturally occurring or synthetic channel elements on function can be evaluated. Using DNA manipulation, entire genes that code for channels/receptors can be knocked out. Specific amino acids in the receptor proteins can be altered by manipulating the DNA encoding the receptor, resulting in a knocked in receptor with specific amino acid substitutions. Enormous strides have been made in understanding the mechanism of action of anesthetic drugs using these genetic methods and assessments of electrophysiology and animal behavior studies.7
Abnormal Action Potentials
A deficiency of calcium ions in the extracellular fluid (hypocalcemia) prevents the sodium channels from closing between action potentials. The resulting continuous leak of sodium contributes to sustained depolarization or repetitive firing of cell membranes (tetany). Conversely, high calcium ion concentrations decrease cell membrane permeability to sodium and thus decrease excitability of nerve membranes. Low potassium ion concentrations in extracellular fluid increase the negativity of the resting membrane potential, resulting in hyperpolarization and decreased cell membrane excitability. Skeletal muscle weakness that accompanies hypokalemia presumably reflects hyperpolarization of skeletal muscle membranes. Local anesthetics decrease permeability of nerve cell membranes to sodium ions, preventing achievement of a threshold potential that is necessary for generation of an action potential. Blockage of cardiac sodium ion channels by local anesthetics may result in altered conduction of cardiac impulses and decreases in myocardial contractility.
Neurotransmitters and Receptors
Neurotransmitters are chemical mediators that are released into the synaptic cleft in response to the arrival of an action potential at the nerve ending. Neurotransmitter release is voltage dependent and requires the influx of calcium ions into the presynaptic terminals (see Fig. 3-2). Synaptic vesicles of the cell body and dendrites of neurons are the sites of continuous synthesis and storage of neurotransmitters. These vesicles may contain and release more than one neurotransmitter. Neurotransmitters may be excitatory or inhibitory, depending on the ion selectivity of the protein receptor. A postsynaptic receptor may be excited or inhibited, reflecting the existence of both types of receptors in the same postsynaptic neuron. Furthermore, the same neurotransmitter may be inhibitory at one site and excitatory at another. This is particularly applicable to G protein–coupled receptors as the associated G protein determines the polarity of the response. Some neurotransmitters function as neuromodulators or coagonists in that they influence the sensitivity of receptors to other neurotransmitters. For example, glycine is an important coagonist at the N-methyl-d-aspartate (NMDA) receptor.
Volatile anesthetics produce a broad spectrum of actions, as reflected by their ability to modify both inhibitory and excitatory neurotransmission at presynaptic and postsynaptic loci within the CNS. The precise mechanism of these effects remains uncertain. It is likely that volatile anesthetics interact with multiple neurotransmitter systems by a variety of mechanisms.8 In general, volatile anesthetics inhibit excitatory receptors (NMDA and nicotinic acetylcholine receptors) and potentiate the action of inhibitory receptors (GABAA and glycine). To quote Ted Eger, “How do they know?” Inhaled anesthetics may depress excitable tissues at all levels of the nervous system by interacting with neuronal membranes,9 resulting in a decreased release of neurotransmitters and transmission of impulses at synapses as well as a general depression of excitatory postsynaptic responsiveness.
The list of chemical mediators functioning as excitatory or inhibitory neurotransmitters continues to increase (Table 3-2). Glutamate is the major excitatory neurotransmitter in the CNS, whereas GABA is the major inhibitory neurotransmitter.8 Acetylcholine, dopamine, histamine, and norepinephrine are widely distributed and play important roles in sleep pathways that are impacted upon by general anesthetics. Neuromodulators coexist in presynaptic terminals with neurotransmitters but do not themselves cause substantive voltage or conductance changes in postsynaptic cell membranes. They can, however, amplify, prolong, decrease, or shorten the postsynaptic response to selected neurotransmitters.

Receptors can be classified by their cellular localization. Receptors on the cell membrane act as signal transducers by binding the extracellular signal molecule and converting this information into an intracellular signal that alters target cell function. Most signaling molecules are hydrophobic and interact with cell surface receptors that are directly or indirectly coupled to effector molecules. There are three classes of cell surface receptors as defined by their signal transduction mechanisms: guanine nucleotide-binding protein (“G protein”) coupled receptors, ligand-gated ion channels, and enzyme-linked transmembrane receptors.
G protein–coupled receptors in the plasma membrane are coupled to specific intracellular G proteins (Fig. 3-6). The binding of the receptor to the ligand activates the G protein, which then activates or inhibits an enzyme, ion channel, or other target. G protein–coupled receptors constitute the largest family of cell surface receptors. A number of different isoforms of G protein subunits (α, β, γ) are present and mediate stimulation or inhibition of functionally diverse effector enzymes and ion channels. Most hormones and many neurotransmitters interact with G protein–coupled cell-surface receptors to produce the cellular response.10–12 The resulting response is often a change in transmembrane voltage and thus neuronal excitability. There is great diversity in the number of G protein–coupled receptors for the same ligand as reflected by multiple receptors for catecholamines and opioids.13

Ligand-gated ion channels are channels in the plasma membrane that respond directly to extracellular ligands, rather than require coupling through G proteins (Fig. 3-7). They are one of three classes of ion channels, the other two being voltage-gated ion channels that respond to transmembrane voltage flux, and “other” gated ion channels that are gated by a huge variety of mechanisms. Rapid synaptic transmission is entirely accomplished through voltage-gated ion channels, which propagate action potentials, and ligand-gated ion channels, which transmit the signal across the synapse.

Enzyme-linked transmembrane receptors are not involved in neuronal signaling per se, as they have relatively slow effects on cells. Most enzyme-linked transmembrane receptors are tyrosine kinases that phosphorylate an intracellular second messenger when the extracellular ligand binds to the receptor (Fig. 3-8). The insulin receptor,14 the atrial natriuretic peptide receptor, and the receptors for many growth factors (nerve growth factor, epidermal growth factor, fibroblast growth factor, and vascular endothelial growth factor) are all examples of tyrosine kinase–linked transmembrane receptors.

There are also intracellular receptors. For example, steroid receptors and thyroid hormone receptors act in the nucleus where they directly regulate the transcription of specific genes, whereas phosphodiesterase inhibitors (e.g., caffeine, milrinone, and sildenafil) act in the cytosol by inhibiting the activity of phosphodiesterase, increasing the cytosolic concentration of cyclic adenosine monophosphate (cAMP). These receptors are also not involved in neuronal signaling per se, because the cellular response is quite slow.
G Protein–Coupled Receptors
G protein–coupled receptors consist of three separate components: a receptor protein, three G proteins (α, β, and γ), and an effector mechanism (see Fig. 3-6). The recognition site faces the exterior of the cell membrane to facilitate access of water-soluble endogenous ligands and exogenous drugs, whereas the catalytic site faces the interior of the cell. There are at least 16 Gα, 5 Gβ, and 11 Gγ proteins,15 providing G protein–coupled receptors that mediate an enormous variety of cellular effects.
The G protein–coupled receptor consists of a single protein with seven transmembrane spanning domains (Fig. 3-9). Binding of an extracellular ligand to the G protein–coupled receptor triggers a conformational change of the protein. That change causes activation of the Gα protein coupled to the interior portion of the receptor. The activation occurs by exchanging a guanine diphosphate (GDP) moiety that is bound to the protein for a guanine triphosphate (GTP) moiety. The activated Gα protein is liberated, where it interacts as a “second messenger” with other proteins in the cell.11 When the Gα protein finds its target, the GTP is hydrolyzed to GDP, and the energy liberated by that hydrolysis powers the effect of the Gα protein on the target protein.

Gα proteins can either be stimulatory, promoting a specific enzymatic reaction within the cell, or inhibitory, depressing a specific enzymatic reaction. For example, β-adrenergic receptors couple with stimulatory Gαs proteins and increase the activity of adenylyl cyclase (also called adenylate cyclase). Opioid receptors associate with inhibitory Gαi proteins that decrease the activity of adenylyl cyclase. By regulating the level of activity of adenylyl cyclase, the β-adrenergic and opioid receptors modulate the internal level of cAMP, which functions as an intercellular second messenger (see Fig. 3-6).
Just as Gαs and Gαi modulate adenylyl cyclase, other types of Ga proteins modulate other specific cellular targets. In some cases, the message is transmitted via Gβγ rather than Gα, as described below for G protein regulation of potassium channels.
Many hormones and drugs act through G protein–coupled receptors, including catecholamines, opioids, anticholinergics, and antihistamines. In contrast to the immediate cellular responses associated with ion channels, signals that use G protein–coupled receptors are involved in functions that operate with time courses of seconds to minutes. Some ion channels are also gated by G proteins. These are discussed below with the ion channels.
Dopamine
Dopamine represents more than 50% of the CNS content of catecholamines, with high concentrations in the basal ganglia. Dopamine can be either inhibitory or excitatory, depending on the specific dopaminergic receptor that it activates. Dopamine is important to the reward centers of the brain and plays a key role in addiction and tolerance to anesthetic and analgesic drugs.
Norepinephrine
Norepinephrine is present in large amounts in the reticular activating system and the hypothalamus, where it plays a key role in natural sleep and analgesia. Neurons responding to norepinephrine send excitatory (through α1) and inhibitory (through α2) signals to widespread areas of the brain, including the cerebral cortex. The sedative action of dexmedetomidine is mediated by activation of α2 adrenergic receptors in the locus ceruleus that inhibit firing of the ventral lateral preoptic nucleus of the hypothalamus (VLPO), an endogenous sleep pathway.16 Descending noradrenergic fibers that project to the dorsal horn of the spinal cord play an important tonic inhibitory role in pain transmission. These pathways are augmented by epidural clonidine for postoperative and intrapartum analgesia.
Substance P
Substance P is an excitatory neurotransmitter coreleased by terminals of pain fibers that synapse in the substantia gelatinosa of the spinal cord. Substance P activates the neurokinin-1 G protein–coupled receptor.
Endorphins
Endorphins are endogenous opioid peptide agonists that are secreted by nerve terminals in the pituitary, thalamus, hypothalamus, brainstem, and spinal cord. Endorphins act through the µ opioid receptor, the same receptor responsible for the effects of administered opioids. Endorphins are secreted after exercise and during pain and anxiety. Endorphins facilitate dopamine release and activate inhibitory pain pathways.
Serotonin
Serotonin (5-HT) is present in high concentrations in the brain, where it acts on both ligand-gated ion channels and G protein–coupled receptors. Serotonin receptors are located in the chemoreceptor trigger zone, where they are inhibited by ondansetron, granisetron, and other common antiemetic drugs
Histamine
Histamine is present in high concentrations in the hypothalamus and the reticular activating system. Histaminergic neurons present in the tuberomammillary nucleus of the hypothalamus are active during the wake cycle. The sleep promoting properties of antihistamine drugs that cross the blood–brain barrier are due to inhibition of H1 G protein–coupled receptors.
Ion Channels
As pointed out earlier, the normal resting membrane potential is −60 to −80 mV, with the interior of the cell negative relative to the extracellular fluid. The lipid bilayer is mostly impermeable to ions, which must pass in and out of the cell through ion-specific channels. If the flux of ions makes the inside of the cell more negative (“hyperpolarized”), then it is harder for the cell to initiate an action potential. If the flux of ions makes the inside of the cell less negative (“depolarized”), then it is easier for the cell to initiate an action potential.
When ion channels open, ions usually flow in the direction favored by their concentration gradient. Extracellular concentrations of sodium, calcium, and chloride greatly exceed intracellular concentrations, and thus these ions flow into cells when the appropriate ion channel opens. Intracellular concentrations of potassium greatly exceed extracellular concentrations, and thus potassium follows out of cells whenever a potassium channel is opened. The inwardly rectifying potassium channel is an exception in that potassium flows into the cell, opposite the concentration gradient, in response to the electrical gradient.
When sodium flows into a cell, it makes the interior less negative. Sodium channels are thus depolarizing. When potassium flows out of a cell, it makes the interior more negative. Therefore, potassium channels are hyperpolarizing. Sodium channels open to conduct action potentials, after which potassium channels open to restore the resting negative potential and terminate the action potential.
When chloride flows into a cell, the interior becomes more negative, or hyperpolarized. Because it is harder for a hyperpolarized cell to initiate an action potential, chloride channels are “inhibitory,” at least after birth. When calcium flows into a cell, the interior becomes less negative, or “depolarized.” Because it is easier for a depolarized cell to initiate an action potential, calcium channels are “excitatory.” Calcium can also act as a second messenger within the cell.
When cell membranes are depolarized (the outside becomes less negative relative to the inside) or the appropriate ligand is present, these ion channels undergo conformational changes, the ion channel opens, and ions pass through. About 104 to 105 ions flow per millisecond per channel and thousands of channels may open during a single action potential.
As mentioned previously, there are three basic types of ion channels: (a) ligand-gated ion channels (ionotropic receptors), (b) voltage-sensitive ion channels, and (c) ion channels that respond to other types of gating.
Ligand-Gated Ion Channels
Ligand-gated ion channels (ionotropic receptors) are complexes of protein subunits that act as switchable portals for ions. Ligand-gated ion channels are involved principally with fast synaptic transmission between excitable cells. Binding of signaling molecules to these receptors causes an immediate conformational change in the ion channels, opening (usually) or closing (rarely) the channel to alter the ion permeability of the plasma membranes and therefore the membrane potential. Ligand-gated ion channels are activated by ligands for which they are named. Nicotinic acetylcholine receptors (nAChRs), serotonin receptors (5-HT3), γ-aminobutyric acid receptors (GABAA) (see Fig. 3-7), and glycine receptors are opened in the presence of acetylcholine, serotonin, GABA, and glycine, respectively. Sometimes the agonist for which the channel is named is not the native agonist. For example, NMDA and α-amino-3-hydroxyl-5-methyl-4-isoxazole-propionate (AMPA) receptors are opened selectively by NMDA and AMPA, but the native agonist for both receptors is glutamate.
Excitatory Ligand-Gated Ion Channels
Excitatory ligand-gated ion channels cause the inside of the cell to become less negative, typically by facilitating the influx of cations into the cell.
Acetylcholine
Acetylcholine is an excitatory neurotransmitter that activates muscarinic and nicotinic receptors in the CNS. Nicotinic acetylcholine receptors are nonspecific cation channels, permitting sodium and in some cases calcium to flow into cells, and potassium to flow out of cells. Because the flow of sodium and calcium is driven both by concentration and electrical gradients, the channel produces a net positive inward flux of cations and is therefore depolarizing (the interior becomes less negative). Nicotinic acetylcholine receptors in the brain are most commonly in a presynaptic location where they act as a “gain control mechanism” to enhance the release of other neurotransmitters. Acetylcholine-releasing neurons play an important role in native sleep pathways where acetylcholine mediates arousal. Although all volatile anesthetics are highly potent inhibitors of the nicotinic acetylcholine receptors that mediate this response,17 direct nicotinic inhibition is not likely responsible for the hypnotic actions of volatile anesthetics. Nicotinic acetylcholine receptors are largely antagonized at volatile anesthetic concentrations; 1/10 of that induce immobility and thus at concentrations associated with a fully awake patient.18,19 Injection of nicotine into the central medial thalamus reversed the hypnotic effect of continued sevoflurane.20 However, in this case, nicotine was acting as an arousing stimulus. Microinfusion of the broad-spectrum nicotinic antagonist mecamylamine did not add to the hypnotic potential of sevoflurane by reducing the dose necessary for hypnosis.
The excitatory effect on the CNS mediated through nicotinic ion channels contrasts with the inhibitory effects that are mediated by the G protein–coupled muscarinic acetylcholine receptors in the peripheral parasympathetic nervous system.
Nicotinic acetylcholine receptors are also responsible for activating muscle contraction. Nondepolarizing muscle relaxants work by blocking the acetylcholine binding site. Because these channels cause depolarization, they are excitatory.
Glutamate
Glutamate is the major excitatory amino acid neurotransmitter in the CNS. Glutamate receptors are nonselective cation channels, permitting sodium and some calcium to flow into cells, and potassium to flow out of cells. Because nonspecific cation channels primarily favor net inward flux of cations down the electrical gradient, glutamate receptors are depolarizing and excitatory. Glutamate-responsive receptors are distributed widely in the CNS. Glutamate plays a key role in learning, and memory, central pain transduction, and pathologic processes such as excitotoxic neuronal injury following CNS trauma or ischemia.
Glutamate is synthesized by the deamination of glutamine via the tricarboxylic acid cycle. Glutamate is released into the synaptic cleft in response to depolarization of the presynaptic nerve terminal. The release of glutamate from presynaptic terminals is a calcium ion-dependent process regulated by multiple types of calcium channels. In common with many other central neurotransmitter systems, the actions of glutamate within the synaptic cleft are terminated by high-affinity sodium-dependent reuptake of glutamate.
The two main subgroups of glutamate receptors are inotropic and metabotropic receptors.8 Ionotropic glutamate receptors (NMDA, AMPA, and kainate receptors) are ligand-gated ion channels. Glutamate receptors that respond to NMDA are associated with neuropathic pain and opioid tolerance and are blocked by ketamine. NMDA receptors are highly calcium permeable. Glutamate receptors that respond to AMPA and kainate are involved with fast synaptic transmission and synaptic plasticity, including long-term potentiation.
Metabotropic glutamate receptors are transmembrane receptors that are linked to G proteins that modulate intracellular second messengers such as inositol phosphates and cyclic nucleotides.
Serotonin
The serotonin (5-HT) receptor is also excitatory, permitting passage of sodium, potassium, and calcium cations as described for the nicotinic acetylcholine receptor.
Inhibitory Ligand-Gated Ion Channels
Inhibitory ligand-gated ion channels cause the inside of the cell to become less negative, typically by facilitating the flux of chloride into the cell. Potassium channels that facilitate the efflux of potassium ions are also inhibitory.
γ-Aminobutyric Acid
GABA is the major inhibitory neurotransmitter in the brain. When two molecules of GABA bind to the GABA receptor, the chloride channel in the center of the receptor opens and chloride ions enter the cell following their concentration gradient (see Fig. 3-7).11 The negatively charged chloride ion hyperpolarizes the interior of the cell, rendering GABA receptors inhibitory shortly after birth. It is estimated that as many as one-third of the synapses in the brain are GABAergic. The chloride channel is formed from the α and β subunits, with or without γ and δ subunits.
In the developing brain neurons have higher concentrations of chloride then the extracellular fluid. As a result, opening of the GABA chloride channel initiates a flux of negatively charged chloride ions out of the cell, depolarizing the cell. Later in development, the potassium/chloride cotransporter appears. This transporter decreases intracellular chloride in exchange for extracellular potassium, creating a concentration gradient for chloride that favors inward flux.21 The change in chloride concentration gradient renders the GABA receptor hyperpolarizing and hence inhibitory.
GABA receptors are the target of propofol, etomidate, and thiopental, which can directly open the channel at high concentration, or at lower concentration increase sensitivity to exogenous GABA. Benzodiazepines also work through GABA receptors but increase the sensitivity of the receptor to exogenous GABA only rather than directly opening the ion channel. There is increasing evidence that extrasynaptic GABA receptors are important in volatile anesthetic-induced behavioral responses.
Glycine
Glycine is the principal inhibitory neurotransmitter in the spinal cord, acting through the glycine receptor to increase chloride ion conductance into the cell, causing hyperpolarization. Glycine receptors are also present in the brain. These channels are involved in many neurologic processes and are modulated by a variety of anesthetic drugs but are not known to be responsible for any specific anesthetic induced behavior.
Strychnine and tetanus toxin result in seizures because they antagonize the effects of glycine on postsynaptic inhibition. Visual disturbances after transurethral resection of the prostate in which glycine is the irrigating solution may reflect the role of this substance as an inhibitory neurotransmitter in the retina.22 Amplitude and latency of visual evoked potentials are altered by infusions of glycine.23
Voltage-Gated Ion Channels
Voltage-gated ion channels are complexes of protein subunits that act as switchable portals sensitive to membrane potential through which ions can pass through the cell membrane. They are “voltage-sensitive” because they open and close in response to changes in voltage across cell membranes. Charged portions of the molecule physically move in response to voltage changes to energetically favor the open or closed state of the channel. For example, the sodium channel opens in response to a sudden depolarization, propagating the action potential in nerves. Voltage-gated ion channels are present in neurons, skeletal muscles, and endocrine cells. They are often named based on the ion that passes through the channel (e.g., sodium, chloride, potassium, and calcium channels).
The voltage-gated sodium channel is of particular interest to anesthesiologists, because it is the site of local anesthetic action. Local anesthetics block neural conduction by blocking passage of sodium through the voltage-gated sodium channel.
The human ether-a-go-go related gene (hERG) potassium channel is a voltage-gated inwardly rectifying potassium channel, mostly famous for its association with prolonged QT syndrome. The hERG potassium channel is sensitive to many drugs and is responsible for sudden death from drugs that predispose the patient to torsades de point. Inhibition of the hERG potassium channel is also responsible for the U.S. Food and Drug Administration (FDA) black box warning on droperidol.
G Protein–Gated Ion Channels
Some ion channels are directly gated by G proteins (Fig. 3-10). G protein–gated potassium channels are the most well studied of the G protein–regulated ion channels.24 The first identified G protein–regulated ion channel was the cardiac potassium channel, which is directly regulated by the M2 muscarinic acetylcholine G protein–coupled receptor.25 This is one of many inward rectifying potassium channels that share the unusual property of permitting influx of potassium ions into the cell following the electrical gradient, rather than the more typical outward flux of potassium following the ionic concentration gradient. G protein regulated inwardly, rectifying potassium channels, commonly referred to as GIRKs, are regulated by Gβγ rather than Gα. In addition to acetylcholine, A1 adenosine, α2 adrenergic, D2 dopamine, opioid, serotonin, and GABAB receptors are coupled directly to GIRKs.24,26

Other Gated Ion Channels
Other types of ion channel gating include gating by other ions (e.g., hydrogen, calcium), second messengers (e.g., cAMP, cyclic guanosine monophosphate [cGMP]), and tissue injury (acid, stretch, temperature, cytokines).
Receptor Concentration
Receptors in cell membranes are not static components of cells. Excess circulating concentrations of ligand often results in a decrease in the density of the target receptors in cell membranes. For example, the excessive circulating norepinephrine in patients with pheochromocytoma leads to downregulation of β-adrenergic receptors. Desensitization of receptor responsiveness is the waning of a physiologic response over time despite (and, caused by) the presence of a constant stimulus.12 Drug-induced antagonism of receptors often results in an increased density of receptors in cell membranes (upregulation). Abrupt discontinuation of the antagonist can result in an exaggerated response to the endogenous agonist. This is one reason that most cardiovascular medicines should be continued throughout the perioperative period.
Receptor Diseases
Numerous diseases are associated with receptor dysfunction. For example, failure of parathyroid hormone and arginine vasopressin to produce increases in cAMP in target organs manifests as pseudohypoparathyroidism and nephrogenic diabetes insipidus, respectively. Grave’s disease and myasthenia gravis reflect development of antibodies against thyroid-stimulating hormone and nicotinic acetylcholine receptors, respectively.
The Synapse
Structure
The synapse functions as a diode that transmits an action potential from the presynaptic membrane to the postsynaptic membrane across the synaptic cleft (Fig. 3-11). The presynaptic membrane contains the vesicles of neurotransmitter and the reuptake pump that returns the neurotransmitter to the presynaptic axoplasm following neurotransmitter release. It also contains the voltage-gated calcium channel. Synaptic transmission starts when an afferent action potential arrives at the voltage-gated calcium channel.

The depolarization permits the influx of calcium ions through the voltage-gated calcium channel. Calcium ions bind to specialized proteins called the release apparatus on axonal and vesicular membranes. Calcium triggers the fusion of the vesicle to the cell membrane and the release of the neurotransmitter into the synaptic cleft through exocytosis, resulting in the extrusion of the contents of the synaptic vesicles. Calcium in the extracellular fluid is essential to the release of neurotransmitters in response to an action potential. The effect of calcium is antagonized by magnesium.
The neurotransmitter in the cleft binds to receptors in the postsynaptic membrane. This binding initiates an efferent action potential in the dendrite of the efferent nerve, which is then propagated. Immediately behind the postsynaptic membrane is the postsynaptic density. The postsynaptic density contains a variety of receptors and structural proteins responsible for maintaining synapse homeostasis.
There are several common misconceptions conveyed by the usual representation of the synapse. First, Figure 3-11 suggests that the synapse consists of two distinct plug-shaped entities that are joined together to form a synapse. Often, the presynaptic neuron may be no more than a slight widening of the axon, the “synaptic varicosity” or “bouton,” because of the presence of the vesicles containing the neurotransmitter. Second, the synapse often appears as a wide gap, as in Figure 3-11. However, the synapse is extremely narrow, on the order of just 20 nm, as shown in Figure 3-12. When the vesicle releases its content into the synapse, the concentration of neurotransmitter is extraordinarily high for a very brief period of time. Lastly, both dendrites and axons have extensive arborizations. The interconnection of hundreds of arborizations across tens of billions of brain cells creates circuits of unimaginable complexity.

Synaptic Modulation
The resting transmembrane potential of neurons in the CNS is about −70 mV, less than the −90 mV in large peripheral nerve fibers and skeletal muscles. The resting transmembrane potential is important for controlling the responsiveness of neurons and is impacted on by extrasynaptic receptors as well as the sodium-potassium ATP exchanger. Postsynaptic inhibitory and excitatory potentials modulated by synaptic and nonsynaptic signaling pathways sum to determine the likelihood of depolarization in response to an incoming stimulus.
Synaptic Delay
Synaptic delay is the 0.3 to 0.5 millisecond necessary for the transmission of an impulse from the synaptic varicosity to the postsynaptic neuron.27 This synaptic delay reflects the time for release of the neurotransmitter from the synaptic varicosity, diffusion of the neurotransmitter to the postsynaptic receptor, and the subsequent change in permeability of the postsynaptic membrane to various ions.
Synaptic Fatigue
Synaptic fatigue is a decrease in the number of discharges by the postsynaptic membrane when excitatory synapses are repetitively and rapidly stimulated. For example, synaptic fatigue decreases excessive excitability of the brain as may accompany a seizure, thus acting as a protective mechanism against excessive neuronal activity. The mechanism of synaptic fatigue is presumed to be exhaustion of the stores of neurotransmitter in the synaptic vesicles. Synaptic fatigue is unmasked at the neuromuscular junction in myasthenia gravis when the enormous reserve for neuromuscular transmission is limited by either pre- or postsynaptic autoimmune damage.
Posttetanic Facilitation
Posttetanic facilitation is increased responsiveness of the postsynaptic neuron to stimulation after a rest period that was preceded by repetitive stimulation of an excitatory synapse. This phenomenon reflects increased release of neurotransmitters due to enhanced local concentrations of intracellular calcium. Posttetanic facilitation may be a mechanism for short-term memory and sensory neuron wind-up.
Factors that Influence Neuron Responsiveness
Neurons are highly sensitive to changes in the pH of the surrounding interstitial fluids. For example, alkalosis enhances neuron excitability. Voluntary hyperventilation can evoke a seizure in a susceptible individual. Conversely, acidosis depresses neuron excitability, with a decrease in arterial pH to 7.0, potentially causing coma. Hypoxia can cause total refractoriness in neurons within 3 to 5 seconds as reflected by the almost immediate onset of unconsciousness following cessation of cerebral blood flow. This response is in part protective because the metabolic activity of inactive neurons is an order of magnitude less than that of active neurons.
Central Nervous System
The brain, brainstem, and spinal cord constitute the CNS. The brain is a complex collection of neural networks that regulate their own and each other’s activity. Activity within the CNS reflects a balance between excitatory and inhibitory influences, a homeostasis that is normally maintained within relatively narrow limits. Anatomic divisions of the brain reflect the distribution of brain functions ( Fig. 3-13).

The two cerebral hemispheres constitute the cerebral cortex, where sensory, motor, and associational information is processed. The limbic system lies beneath the cerebral cortex and integrates the emotional state with motor and visceral activities. The thalamus lies in the center of the brain beneath the cerebral cortex and basal ganglia and above the hypothalamus. The neurons of the thalamus are arranged in nuclei that act as relays between the incoming sensory pathways and the cerebral cortex, hypothalamus, and basal ganglia. The hypothalamus is the principal integrating region for the autonomic nervous system and regulates other functions, including systemic blood pressure, body temperature, water balance, secretions of the pituitary gland, emotions, and sleep.
The brainstem connects the cerebral cortex to the spinal cord and contains most of the nuclei of the cranial nerves and the reticular activating system. The reticular activating system is essential for regulation of sleep and wakefulness. The cerebellum arises from the posterior pons and is responsible for coordination of movement, maintenance of body posture, and certain types of motor memory.
The spinal cord extends from the medulla oblongata to the lower lumbar vertebrae. Ascending and descending tracts are located within the white matter of the spinal cord, whereas intersegmental connections and synaptic contacts are concentrated in the gray matter. Sensory information flows into the dorsal portion (posterior) of the gray matter, and motor outflow exits from the ventral (anterior) portion. Preganglionic neurons of the autonomic nervous system are found in the intermediolateral portions of the gray matter.
Cerebral Hemispheres
The two cerebral hemispheres, known as the cerebral cortex, constitute the largest division of the human brain. Regions of the cerebral cortex are classified as sensory, motor, visual, auditory, and olfactory, depending on the type of information that is processed. Frontal, temporal, parietal, and occipital designate anatomic positions of the cerebral cortex (Fig. 3-14). For each area of the cerebral cortex, there is a corresponding and connecting area to the thalamus such that stimulation of a small portion of the thalamus activates the corresponding and much larger portion of the cerebral cortex. Indeed, the cerebral cortex is actually an evolutionary outgrowth of the lower regions of the nervous system, especially the thalamus. The functional part of the cerebral cortex is composed mainly of a 2- to 5-mm layer of neurons covering the surface of all the convolutions. It is estimated that the cerebral cortex contains 50 to 100 billion neurons.

Anatomy of the Cerebral Cortex
The sensorimotor cortex is the area of the cerebral cortex responsible for receiving sensation from sensory areas of the body and for controlling body movement (see Fig. 3-14).3 The premotor cortex is important for controlling the functions of the motor cortex. The motor cortex lies anterior to the central sulcus. Its posterior portion is characterized by the presence of large, pyramid-shaped (pyramidal or Betz) cells.
Topographic Areas
The area of the cerebral cortex to which the peripheral sensory signals are projected from the thalamus is designated the somesthetic cortex (see Fig. 3-14).3 Each side of the cerebral cortex receives sensory information exclusively from the opposite side of the body. The size of these areas is directly proportional to the number of specialized sensory receptors in each respective area of the body. For example, a large number of specialized nerve endings are present in the lips and the thumbs, whereas only a few are present in the skin of the trunk.
The motor cortex is organized into topographic areas corresponding to different regions of the skeletal muscles. The spatial organization is similar to that of the sensory cortex. In general, the size of the area in the motor cortex is proportional to the preciseness of the skeletal muscle movement required. As such, the digits, lips, tongue, and vocal cords have large representations in humans. The various topographic areas in the motor cortex were originally determined by electrical stimulation of the brain during local anesthesia and observation of the evoked skeletal muscle response. Such stimulation can be used intraoperatively to identify the location of the motor cortex and thus avoid damage to this area. The motor cortex is commonly damaged by loss of blood supply as occurs during a stroke.
Corpus Callosum
The two hemispheres of the cerebral cortex, with the exception of the anterior portions of the temporal lobes, are connected by fibers in the corpus callosum. The anterior portions of the temporal lobes, including the amygdala, are connected by fibers that pass through the anterior commissure. The corpus callosum and anterior commissure make information processed or stored in one hemisphere available to the other hemisphere.
Dominant versus Nondominant Hemisphere
Language function and interpretation is typically localized in the dominant cerebral hemisphere, whereas spatiotemporal relationships (ability to recognize faces) is localized in the nondominant hemisphere. The left hemisphere is dominant in 90% of right-handed individuals and 70% of left-handed individuals. Destruction of the dominant cerebral hemisphere in adults results in loss of nearly all intellectual function.
The historical failure to document an important role of prefrontal lobes in intellectual function (frontal lobotomy) is surprising because the principal difference between the brains of humans and monkeys is the prominence of human prefrontal areas. It seems that the function of the prefrontal areas in humans is to provide additional cortical area in which thought processing can occur. Furthermore, selection of behavior patterns for different situations may be an important role of the prefrontal areas that transmit signals to the limbic areas of the brain. Persons without prefrontal lobes may react precipitously in response to incoming signals or manifest undue anger at slight provocations. Ability to maintain a sustained level of concentration is lost in the absence of the prefrontal lobes.
Memory
The cerebral cortex, especially the temporal lobes, serves as a storage site for information that is often characterized as memory.28 The mechanisms for short-term and long-term memory are not completely understood but are thought to be encoded through selective synaptic strengthening in response to experience.
Short-Term Memory
The favored explanation for short-term memory is posttetanic potentiation. For example, tetanic stimulation of a synapse for a few seconds causes increased excitability of the synapse that lasts for seconds to hours. This change in excitability of the synapse is mediated by increased local intracellular calcium concentrations that facilitate transmitter release and act as a second messenger to activate genetic programs that result in structural synaptic stabilization.
Long-Term Memory
Long-term memory depends on stable synaptic changes that are induced by experience. The stability of this system is evidenced by total inactivation of the brain by hypothermia or anesthesia without detectable significant loss of long-term memory. Long-term memory is thought to rely on long-term synaptic potentiation mediated by structural changes. Long-term potentiation is the enhanced synaptic transmission observed after repeatedly stimulating a presynaptic neuron. The mechanism often involves increased expression of NMDA receptors and voltage-gated calcium channels in the postsynaptic neuron.29 Thus, protein transcription and synaptic remodeling are an essential component of long-term memory. The hippocampus and amygdala are critically involved in creating new long-term memories. However, long-term memories are not actually stored in the hippocampus and amygdala. Sleep is known to play an important role in the formation of long-term memory.30 However, the actual mechanism by which long-term memories are stored remains a fascinating unsolved puzzle.
Everyone knows from personal experience that repetition is essential to forming long-term memory. There is an old joke about a man asking a fellow pedestrian in New York, “How do you get to Carnegie Hall?” The pedestrian replies, “Practice, practice, practice.” It has been repeatedly demonstrated in animal studies as well that repetition is key to forming long-term memories. Long-term potentiation is the synaptic consequence of repeated stimulation, which is one reason that long-term potentiation is thought to be the fundamental building block of long-term memory.
We also know that memories are transferred from short-term memory to long-term memory. Because the creation of long-term memory requires anatomic changes in the synapse, this transfer requires time. This suggests, and studies confirm, that if the brain is not given adequate time to make this transfer, there will be no transfer from short-term memory to long-term memory. This has direct applicability to the practice of anesthesia. During the provision of general anesthesia, we are vigilant for signs of inadequate anesthesia and intraoperative awareness (discussed further at the end of this section). If a patient has conscious perception of the surgery, this will initially be part of the patient’s short-term memory. Rapid deepening of the anesthesia, for example by administering a bolus of propofol in response to patient movement, will prevent transfer of the recall from short-term memory to the long-term memory, and the patient will be amnestic. Conversely, if the patient is paralyzed and is awake for many minutes without the anesthesiologist being aware of the situation, then there has been adequate time for transfer of the short-term memory to long-term memory.
Because the neural substrate of memory is not well understood, memory is often discussed from a psychological point of view. Memories typically involve multiple senses (sight, hearing, touch), emotions (fear, satisfaction, pleasure, anger), and cognitive assessment (“I remember thinking that. . .”). These are thought to be held together in a facilitated circuit that has been called a memory engram or memory trace. Initially the circuit is facilitated through posttetanic potentiation in short-term memory. If memory is to persist, this is replaced with long-term potentiation. The pieces of the engram are consolidated through hypothalamic circuitry. The memory engram is reinforced with every subsequent recall of the memory. An important feature of the process of consolidation is that long-term memory is encoded into different categories. New memories are not stored randomly in the brain but seem to be associated with previously encoded and similar information. This permits scanning of memory to retrieve desired information at a later date. We also know that memory scanning is often a subconscious process. This is confirmed by the daily experience of struggling to recall a fact or event, only to have the memory suddenly jump into our consciousness hours later.
Postoperative Cognitive Dysfunction
Postoperative cognitive dysfunction (impaired memory) persisting after 3 months has been described in 10% of elderly patients receiving general anesthesia without known arterial hypoxemia or systemic hypotension.31 Inhaled anesthetics are known to alter the proteins involved in the formation of Alzheimer’s disease.32 It is unclear whether the postoperative cognitive dysfunction is caused by anesthetic injury to the aged brain, as might be caused by increasing the polymerization of β amyloid, or is caused by the combined effects of surgical trauma, inflammation, social interruption, anesthesia, and other unidentified causes.
Awareness and Recall during Anesthesia
Awareness, defined as conscious memory of events during anesthesia, has been a recurrent problem particularly since the introduction of neuromuscular-blocking drugs.33 Neuromuscular blocking drugs permit inadequate anesthesia to be administered without obvious patient withdrawal from the noxious stimulus. The use of neuromuscular blockade is a risk factor for awareness under general anesthesia, particularly awareness that is associated with memories of pain and complicated by posttraumatic stress disorder.34
Memory may be considered to be conscious (explicit) or unconscious (implicit). Conscious memory includes spontaneous recall and recognition memory. Unconscious memory is manifest by altered performance or behavior due to experiences that are not consciously remembered. By definition, general anesthesia abolishes conscious memory, but the extent to which it also abolishes unconscious memory is controversial. Behavioral disturbances manifest as night terrors in children after anesthesia may be an expression of implicit memory in the dream state.
The incidence of awareness with recall (conscious memory) following general anesthesia has been estimated at between 1 and 5 in 1,000 general anesthetics, depending on the risk group.35–37 Although the incidence of conscious recall of intraoperative events is rare and the development of posttraumatic stress disorder is even more uncommon, the fact that approximately 20 million general anesthetics are administered annually in the United States would correspond to 26,000 cases of awareness (0.13% of approximately 20 million) each year. The incidence of awareness in patients undergoing cesarean section was 0.4% and for cardiac surgery was 1.14% to 1.50% .38,39 A higher incidence of awareness has been described for major trauma cases (11% to 43%) where the concentration of anesthetic administered is limited by hemodynamic instability.40 Many cases of conscious awareness during surgery can be attributed to intentionally or unintentionally low concentrations of administered anesthetic.
Subanesthetic doses of inhaled anesthetics have powerful inhibitory effects on short-term memory, and the decrease in the transfer of information from the periphery to the cerebral cortex associated with general anesthesia prevents the recall of intraoperative events.8
Isoflurane (and presumably other volatile anesthetics) and nitrous oxide suppress memory in a dose-dependent manner, and isoflurane is more potent than equivalent concentrations of nitrous oxide (Fig. 3-15).41 For example, conscious memory was prevented by 0.45 minimum alveolar concentration to prevent movement (MAC) isoflurane or 0.6 MAC nitrous oxide. Isoflurane concentrations of ≥0.6 MAC prevent conscious recall and unconscious learning of factual information and behavioral suggestions.42

Recognizing Awareness
Monitoring patients during general anesthesia for the presence of awareness is challenging. Despite a variety of monitoring methods, awareness may be difficult to recognize in real time. Indicators of awareness (heart rate, blood pressure, and skeletal muscle movement) are often masked by anesthetic and adjuvant drugs (β-adrenergic blockers and/or neuromuscular-blocking drugs). Several different monitors, based on analysis of electroencephalogram (EEG) and somatosensory evoked potential patterns, have been introduced in hopes of addressing this issue.
Brainstem
Homeostatic life-sustaining processes are controlled subconsciously in the brainstem. Examples of subconscious activities of the body regulated by the brainstem include control of systemic blood pressure and breathing in the medulla. The thalamus serves as a relay station for most afferent impulses before they are transmitted to the cerebral cortex. The hypothalamus receives fibers from the thalamus and is also closely modulated by the cerebral cortex.
Limbic System and Hypothalamus
Behavior associated with emotions is primarily a function of structures known as the limbic system (hippocampus, basal ganglia) located in the basal regions of the brain. The hypothalamus functions in many of the same roles as the limbic system and is considered by some to be part of the limbic system rather than a separate structure. In addition, the hypothalamus controls many internal conditions of the body, such as core temperature, thirst, and appetite. The great Oxford neurophysiologist Sir Charles Sherrington called the hypothalamus the head ganglion of the autonomic nervous system. The suprachiasmatic nucleus of the hypothalamus helps to maintain the body clock by secreting melatonin and other mediators according to the circadian rhythm. This nucleus sits just above the optic chiasm and receives inputs from the optic nerve that serve to entrain the circadian rhythm to environmental light. At high doses, melatonin and its analogs have properties similar to a general anesthetic.43
Basal Ganglia
The basal ganglia include the caudate nucleus, putamen, globus pallidus, substantia nigra, and subthalamic nucleus. Many of the impulses from basal ganglia are inhibitory mediated by dopamine and GABA. The balance between agonist and antagonist skeletal muscle contractions is an important role of the basal ganglia. A general effect of diffuse excitation of the basal ganglia is inhibition of skeletal muscles, reflecting transmission of inhibitory signals from the basal ganglia to both the motor cortex and the lower brainstem. Therefore, whenever destruction of the basal ganglia occurs, there is associated skeletal muscle rigidity. For example, damage to the caudate and putamen nuclei that normally secrete GABA results in choreiform random and continuous uncontrolled movements. Destruction of the substantia nigra and loss of dopaminergic neurons results in a predominance of the excitatory neurotransmitter acetylcholine, manifesting as the skeletal muscle rigidity of Parkinson’s disease. As such, dopamine precursors or anticholinergic drugs are used in the treatment of Parkinson’s disease in an attempt to restore the balance between excitatory and inhibitory impulses traveling from the basal ganglia.
Reticular Activating System
The reticular activating system is a polysynaptic pathway that is intimately concerned with electrical activity of the cerebral cortex. Neurons of the reticular activating system are both excitatory and inhibitory. The reticular activating system determines the overall level of CNS activity, including nuclei important in determining wakefulness and sleep. Selective activation of certain areas of the cerebral cortex by the reticular activating system is crucial for the direction of the attention of certain aspects of mental activity. It is likely that many injected and inhaled anesthetics exert their sedative effects through interaction with the brainstem and midbrain nuclei that mediate arousal and sleep.44 This is not to say that general anesthesia is equivalent to sleep. Although the EEG response to many anesthetics resembles deep slow-wave sleep, a key difference is that afferent stimulation does not cause arousal.
Slow-Wave Sleep
Most of the sleep that occurs each night is slow-wave sleep. The EEG is characterized by the presence of high-voltage δ waves occurring at a frequency of <4 cycles per second. Presumably, decreased activity of the reticular activating system that accompanies sleep permits an unmasking of this inherent rhythm in the cerebral cortex. Slow-wave sleep is restful and devoid of dreams. During slow-wave sleep, sympathetic nervous system activity decreases, parasympathetic nervous system activity increases, and skeletal muscle tone is greatly decreased. As a result, there is a 10% to 30% decrease in systemic blood pressure, heart rate, breathing frequency, and basal metabolic rate.
Desynchronized Sleep
Periods of desynchronized sleep typically occur for 5 to 20 minutes during each 90 minutes of sleep. These periods tend to be shortest when the person is extremely tired. This form of sleep is characterized by active dreaming, irregular heart rate and breathing, and a desynchronized pattern of low-voltage β waves on the EEG similar to those that occur during wakefulness. This brain wave pattern emphasizes that desynchronized sleep is associated with an active cerebral cortex, but this activity does not permit persons to be aware of their surroundings and thus be awake. Despite the inhibition of skeletal muscle activity, the eyes are an exception, exhibiting rapid movements. For this reason, desynchronized sleep is also referred to as paradoxical sleep or rapid eye movement (REM) sleep.
Cerebellum
The cerebellum operates subconsciously to monitor and elicit corrective responses in motor activity caused by stimulation of other parts of the brain and spinal cord. Rapid repetitive skeletal muscle activities, such as typing, playing musical instruments, and running, require intact function of the cerebellum. Loss of function of the cerebellum causes incoordination of fine skeletal muscle activities even though paralysis of the skeletal muscles does not occur. The cerebellum is also important in the maintenance of equilibrium and postural adjustments of the body. For example, sensory signals are transmitted to the cerebellum from receptors in muscle spindles, Golgi tendon organs, and receptors in skin joints. These spinocerebellar pathways can transmit impulses at velocities exceeding 100 m per second, which is the most rapid conduction of any pathway in the CNS. This extremely rapid conduction is important for instantaneous appraisal by the cerebellum of changes that take place in the positional status of the body.
Dysfunction of the Cerebellum
In the absence of cerebellar function, a person cannot predict prospectively how far movements will go. This results in overshoot of the intended mark (past pointing). This overshoot is known as dysmetria, and the resulting incoordinate movements are called ataxia. Dysarthria is present when rapid and orderly succession of skeletal muscle movements of the larynx, mouth, and chest do not occur. Failure of the cerebellum to dampen skeletal muscle movements results in intention tremor when a person performs a voluntary act. Cerebellar nystagmus is associated with loss of equilibrium, presumably because of dysfunction of the pathways that pass through the cerebellum from the semicircular canals. In the presence of cerebellar disease, a person is unable to activate antagonist skeletal muscles that prevent a certain portion of the body from moving unexpectedly in an unwanted direction. For example, a person’s arm that was previously contracted but restrained by another person will move back rapidly when it is released rather than automatically remain in place.
Spinal Cord
The spinal cord extends from the medulla oblongata to the lower border of the first and, occasionally, the second lumbar vertebra. Below the spinal cord, the vertebral canal is filled by the roots of the lumbar and sacral nerves, which are collectively known as the cauda equina. The spinal cord is composed of gray and white matter, spinal nerves, and covering membranes.
Gray Matter
The gray matter of the spinal cord functions as the initial processor of incoming sensory signals from peripheral somatic receptors and as a relay station to send these signals to the brain.
In addition, this area of the spinal cord is the site for final processing of motor signals that are being transmitted downward from the brain to skeletal muscles. Anatomically, the gray matter of the spinal cord is divided into anterior, lateral, and dorsal horns consisting of nine separate laminae that are H-shaped when viewed in cross-section (Fig. 3-16). The anterior horn is the location of α and γ motor neurons that give rise to nerve fibers that leave the spinal cord via the anterior (ventral) nerve roots and innervate skeletal muscles. Cells of Renshaw are intermediary neurons in the anterior horn, providing nerve fibers that synapse in the gray matter with anterior motor neurons. These cells inhibit the action of anterior motor neurons to limit excessive activity. Cells of the preganglionic neurons of the sympathetic nervous system are located lateral to the thoracolumbar portions of the spinal cord. Cells of the intermediate neurons located in the portion of the dorsal horns of the spinal cord known as the substantia gelatinosa (laminae II to III) transmit afferent tactile, temperature, and pain impulses to the spinothalamic tract. The dorsal horn serves as a gate where impulses in sensory nerve fibers are translated into impulses in ascending tracts. There is evidence for a form of memory in the dorsal horn of the spinal cord that is evoked by intense stimulation. Resulting increases in intracellular calcium set into motion long-lasting changes that are associated with central sensitization and result in increased sensitivity to subsequent inoffensive stimuli.

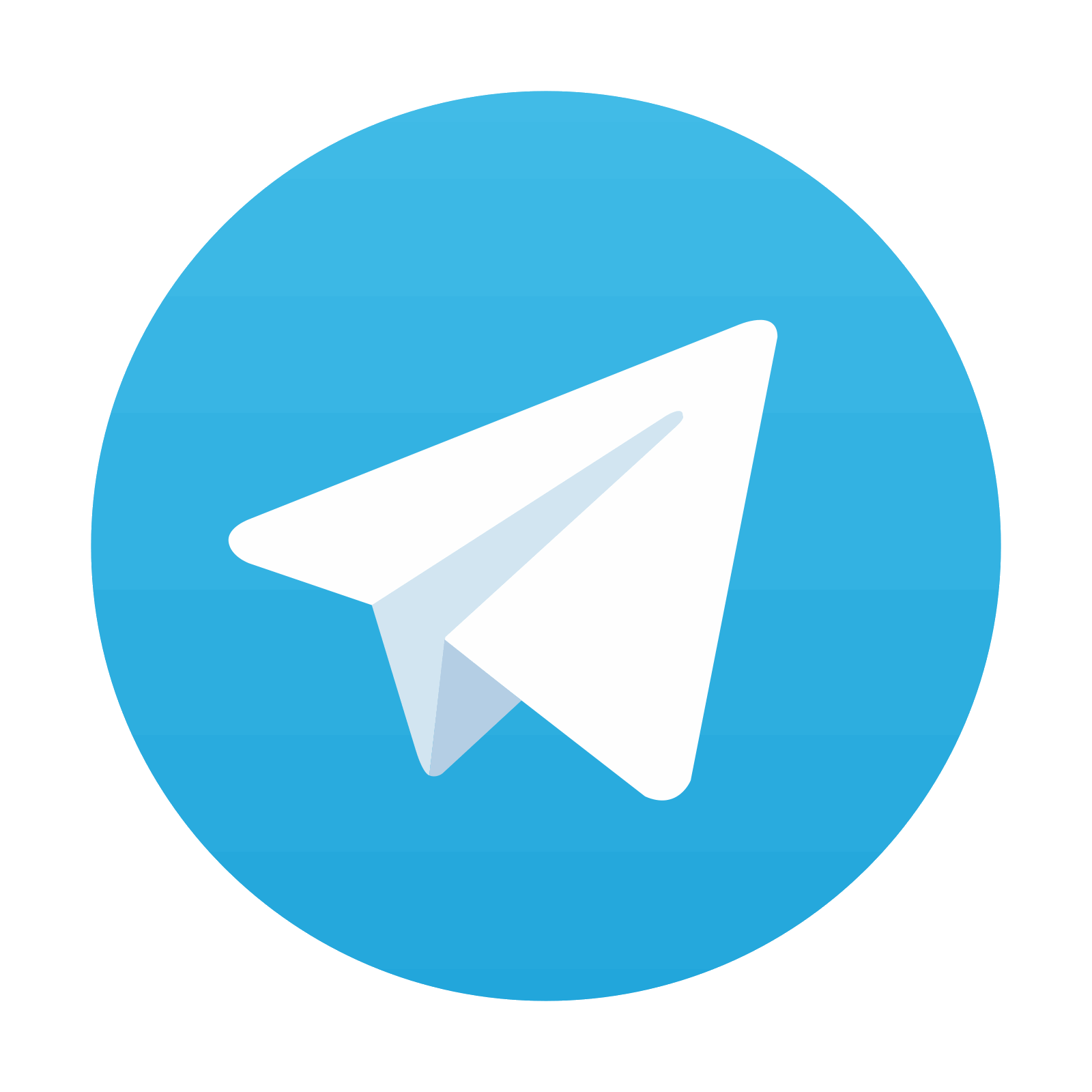
Stay updated, free articles. Join our Telegram channel
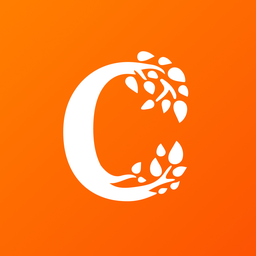
Full access? Get Clinical Tree
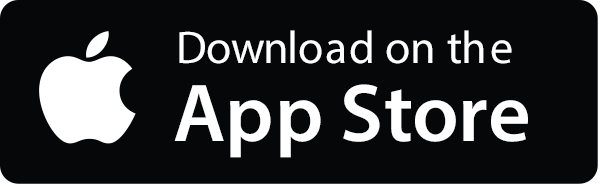
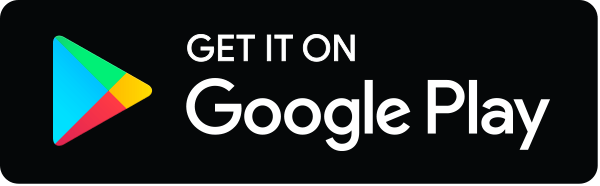