Abstract
Neuromuscular transmission in skeletal muscle occurs when acetylcholine released from the nerve ending binds to the endplate nicotinic acetylcholine receptors (AChRs) on the postjunctional muscle membrane. These AChRs respond by opening channels for the influx of sodium ions into the muscle leading to endplate depolarization and muscle contraction. Activation is terminated when acetylcholine is hydrolyzed by acetylcholinesterase.
Neuromuscular transmission is a complex and dynamic process in which the effects of drugs are composites of actions that vary with drug, dose, activity at the junction and muscle, presence of other drugs, age and concomitant disease. The neuromuscular junction undergo constant structural remodeling influenced by multiple factors including endogenous mediators/hormones, age, and genetic and/or pathologic state.
The etiology of neuromuscular disorders can be classified as inherited or acquired, and anatomically as presynaptic, synaptic, or postsynaptic. They are also classified as autoimmune, congenital, pharmacologic, toxic, or pathologic.
The common defect of all disorders of the neuromuscular junction is a decrease in the margin of safety for neuromuscular transmission, which can fail at any point from the motor neuron to nerve terminal to muscle endplate (synapse) or the muscle itself. If the endplate potential fails to reach the threshold for muscle fiber depolarization, an action potential is not generated, the muscle fiber does not contract, and neurotransmission fails.
In some pathologic states such as denervation, burns, immobilization, inflammation, or sepsis, there is upregulation of immature AChRs and α7-AChRs leading to susceptibility to succinylcholine-induced hyperkalemia and also resistance to the effects of nondepolarizing neuromuscular blockers. Use of neuromuscular blockers during critical illness has both beneficial and deleterious effects; guidelines for their use in the perioperative period need consensus and refinement.
Keywords
Acetylcholine, Acetylcholine receptor, Acetylcholinesterase, Congenital neuromuscular diseases, Neuromuscular junction, Postjunctional receptors, Prejunctional receptors, Train-of-four fade
Chapter Outline
Overview of Neuromuscular Transmission
Structure and Function of the Neuromuscular Junction
Quantal Theory of Neuromuscular Transmission
Synaptic Vesicles and Recycling
Nerve Terminal Action Potential
Plasticity of the Neuromuscular Junction
Age-Associated Changes in the Neuromuscular Junction
Clinical Implications—Actions of Neuromuscular Blocking Drugs
Reversal (Antagonism) of Neuromuscular Blocking Drugs
Nonclassic Actions of Drugs Acting at the Neuromuscular Junction
Neuromuscular Junction in Disease and Injury
The neuromuscular junction (NMJ) is among the most studied of all synapses and serves as a prototype for understanding communication between neurons and effector cells within the central and peripheral nervous systems. Complete understanding of the development and function of the NMJ in normal and pathologic states continues to evolve. The introduction of new and powerful research techniques has revealed detailed information about the mechanics of neuromuscular transmission. Recent discoveries suggest a more complex signaling system with adaptive receptor physiology and a multifaceted action-response scheme. These discoveries, although adding complexity, help align experimentally derived findings with clinical observations of therapeutic effects and side effects of drugs acting on the cholinergic system.
The physiology and pharmacology of the NMJ is pivotal to many areas of anesthetic practice, including perioperative management and critical care. Despite the central role of the NMJ, in many clinical circumstances our ability to manipulate the NMJ to the patient’s advantage is deficient. This is best illustrated by the ongoing problem of residual neuromuscular paralysis recorded after general anesthesia despite the use and availability of intermediate-acting neuromuscular blockers (NMBs), better monitors for assessment of paralysis, and availability of drugs for reversal of paralysis (see Chapter 22 ).
The complexities of normal neuromuscular function are altered by many pathologic states not originally thought to involve the NMJ. For example, an unexpected consequence of improved survival after a critical illness, such as sepsis or acute respiratory distress syndrome (ARDS), is the “unmasking” of profound disease of the nerve, NMJ, and muscle causing substantial morbidity and mortality.
This chapter reviews salient features of the structure and function of the NMJ relevant to understanding the clinical effects of NMBs, neuromuscular monitoring, and the management of disorders of the neuromuscular system in anesthesia and critical care. A detailed discussion of NMB sand drugs that reverse the effects of NMBs is provided in Chapter 22 .
Overview of Neuromuscular Transmission
The classic model of neuromuscular transmission is straightforward. The nerve synthesizes acetylcholine (ACh), which is stored in small uniformly sized membrane vesicles called synaptic vesicles. When the distal motor nerve is stimulated by an action potential, it causes voltage-gated calcium ion (Ca 2+ ) channels to open, giving rise to an abrupt, rapid, transient increase in nerve terminal Ca 2+ concentration. The elevated Ca 2+ concentration triggers fusion of ACh-containing synaptic vesicles to the presynaptic membrane, where they release ACh into the synaptic cleft that separates the nerve from the muscle. ACh released into the cleft binds to nicotinic acetylcholine receptors (AChRs) on the surface of the postjunctional muscle membrane. These heteropentameric ligand-gated ion channels are nonselective caption channels in the postjunctional membrane that allow influx of Na + , resulting in endplate depolarization and muscle contraction. Much of the released ACh is instantaneously hydrolyzed by synaptic acetylcholinesterase present in the cleft and never reaches its target. The excess release of ACh contributes to a margin of safety that ensures successful neurotransmission.
Structure and Function of the Neuromuscular Junction
The structure and function of the NMJ can be conveniently divided into presynaptic, synaptic cleft, and postsynaptic entities ( Fig. 21.1 ).

Presynaptic Structure and Function
The presynaptic portion of the NMJ is responsible for neurotransmitter (ACh) synthesis, uptake and storage of neurotransmitter into synaptic vesicles, ACh release and reuptake of choline after its hydrolysis, and control of ion flow across the nerve terminal cell membrane. Each motor neuron represents a large myelinated axon that extends from the ventral horn of the spinal cord or medulla to the NMJ. As it approaches the target muscle, the myelinated motor axon divides into 20 to 100 unmyelinated fibers, each of which innervates a single muscle fiber. The functional group of terminal nerve fibers and the muscle fibers they serve is called a motor unit . The terminal myelin-free portion of the nerve axon is encapsulated by a Schwann cell. Previously the Schwann cells were thought not to be involved in neuromuscular transmission but were considered to have a support function between nerve and muscle and promote maintenance of the nerve terminal (see Fig. 21.1 ). More recently, however, the terminal Schwann cells, which are glial cells, importantly contribute to presynaptic and postsynaptic maturation. This intricate dance a trois (among terminal Schwann cell, nerve terminal, and muscle) is integral to the efficient communication between the highly concentrated postsynaptic AChRs and perfectly aligned presynaptic ACh release sites.
Synaptic Cleft Structure and Function
The junctional or synaptic cleft is the gap (~50 nm) between the nerve terminal ending and the muscle membrane. The cleft contains a basal lamina composed of a variety of cell and other adhesion macromolecules that modulate neuromuscular signaling processes. These molecules include acetylcholinesterase, α- and β-laminin, and collagen.
After release from the nerve terminal, ACh diffuses the short distance across the synaptic cleft to the postsynaptic membrane. The high concentration of ACh in combination with the short distance between the site of release and the postsynaptic membrane ensures rapid transmission. Approximately 50% of the released ACh is either degraded by acetylcholinesterase or diffuses out of the cleft before it reaches its target. Acetylcholinesterase is anchored to the basal lamina, where it degrades ACh into acetate and choline, thereby terminating the activity of the transmitter. Choline is then taken up into the presynaptic terminal by a specific transporter for resynthesis of ACh within the nerve terminal.
Postsynaptic Membrane
The muscle membrane is highly corrugated with deep invaginations representing primary (shallower) and secondary (deeper) clefts such that the total surface area of each endplate is very large (see Fig. 21.1 ). The depths of these folds vary between muscle types and species. The shoulders of the folds contain high densities of AChRs (~ 5 million in each junction) anchored into the muscle cell membrane by a complex system of cytoskeletal proteins. AChRs are sparse in the depths between the folds, which contain a high density of voltage-gated Na + channels for amplification of AChR-induced depolarization.
The perijunctional zone is the area of muscle that lies immediately beyond the junctional area. It serves the critical function of transducing the signal from the junction into deeper regions of the muscle cell. The perijunctional zone contains a high density of Na + channels that promote amplification of the transduced signal, culminating in muscle contraction. The density of Na + channels in the perijunctional zone is greater than in more distal parts of the muscle membrane. Furthermore, specific isoforms of AChRs and Na + channels are expressed in the perijunctional area at different stages of life and during pathologic conditions that decrease nerve activity. Mutations in AChRs and Na + and Ca 2+ channels in this area have been identified. For example, in hypokalemic periodic paralysis, characterized by episodes of severe flaccid muscle paralysis, the muscle fiber membrane becomes electrically inexcitable, which can be precipitated by low serum K + levels. This pathologic state is the archetypal skeletal muscle channelopathy caused by dysfunction of specific sarcolemmal Na + or Ca 2+ ion channels. These variations in receptors and channels contribute to differences in response to neuromuscular drugs observed in different age groups and pathologic conditions.
Quantal Theory of Neuromuscular Transmission
Synaptic vesicles containing neurotransmitter are clustered along thickened, electron-dense patches of membrane in the terminal portion of the nerve referred to as active zones. High-resolution scanning microscope imaging reveals small protein particles arranged between vesicles in the active zone that are believed to be voltage-gated Ca 2+ channels. These channels permit Ca 2+ to enter the nerve and initiate the process that ultimately triggers vesicle release. The close proximity of voltage-gated Ca 2+ channels to the vesicle fusion apparatus is believed to contribute to the rapidity with which ACh is released (~200 ns).
The number of quanta of ACh released by a stimulated nerve is strongly influenced by the concentration of extracellular ionized Ca 2+ and its influx into the nerve. Doubling extracellular Ca 2+ concentration results in a 16-fold increase in quantal release. The effect of increasing Ca 2+ in the nerve ending is seen clinically as the phenomenon of posttetanic potentiation. This can occur after the nerve of a patient paralyzed with a nondepolarizing NMBs is stimulated at high tetanic frequencies. With each stimulus, Ca 2+ enters the nerve, but because it cannot be excreted as quickly as the nerve is stimulated, it accumulates during the tetanic period. The nerve ending contains an elevated amount of Ca 2+ for some time after the tetanic stimulation, and if another stimulus is applied during this time, it increases the amount of ACh that is released. The increased amount of ACh released antagonizes the nondepolarizing NMBs and results in the characteristic increase in twitch size observed after tetanic stimulation (posttetanic potentiation).
The amount of ACh released by each nerve impulse is large, at least 200 quanta, consisting of about 5000 ACh molecules each, and about 500,000 AChRs are activated. The ions (mainly sodium [Na + ] and some Ca 2+ ) that flow through the AChR channels cause maximal depolarization of the endplate, resulting in an endplate potential that exceeds the threshold for stimulation of the muscle. This produces a biologic advantage. By releasing more molecules of transmitter than actually are needed and evoking a greater response than needed while using only a small fraction of the vesicles and receptors available, neurotransmission has a substantial margin of safety and at the same time has substantial capacity in reserve.
Synaptic Vesicles and Recycling
Two major pools of vesicles are involved in ACh release: a readily releasable pool (sometimes called V2) and a reserve pool (sometimes called V1). The readily releasable pool is smaller and is confined to an area very close to the nerve membrane where the vesicles are bound to the active zones. These are the vesicles that are normally available to release neurotransmitter. The reserve pool contains the majority of synaptic vesicles, which are tethered to the cytoskeleton in a filamentous network composed mainly of actin, synapsin, synaptotagmin, and spectrin.
The larger reserve pool (V1) can be mobilized to the readily releasable store when there is increased demand, such as when the nerve is stimulated at high frequencies or for long periods of time. Under such high demand conditions, increases in Ca 2+ concentration penetrate more deeply than normal into the nerve or might enter via L-type Ca 2+ channels (see later text). This probably activates Ca 2+ -dependent enzymes that break the links with synapsin that hold the reserve vesicles to the cytoskeleton, thereby allowing mobilization of vesicles to release sites. Repeated stimulation of the nerve requires replenishment of the nerve ending with synaptic vesicles, a process known as mobilization .
The aggregate process is involved in maintaining the capacity of the nerve ending to release neurotransmitter. This process encompasses everything from uptake of choline and synthesis of acetate to the movement of vesicles to release sites. The rate-limiting steps of this process appear to be the uptake of choline and the activity of choline acetyltransferase, the enzyme that synthesizes acetylcholine.
The complex process of docking, fusion, and release of neurotransmitter from synaptic vesicles is called exocytosis . Three proteins, synaptobrevin, syntaxin, and synaptosomal-associated protein 25 (SNAP-25), within the group of soluble N-ethylmaleimide-sensitive factor attachment receptor (SNARE) proteins have key roles in the process of exocytosis ( Fig. 21.2 ). A complex of syntaxin and SNAP-25 is attached to the plasma membrane. After initial contact, synaptobrevin on the vesicle forms a ternary complex with syntaxin and SNAP-25 that consists of a zippered helical bundle. This complex forces the vesicle close to the nerve terminal membrane at the active zone (release site). Synaptotagmin, a protein on the vesicular membrane that acts as the Ca 2+ sensor, localizes the vesicles to synaptic zones rich in Ca 2+ channels and stabilizes the vesicles in the docked state. After fusion and exocytosis, the used vesicle and membrane parts are recycled through an active process into the nerve terminal; there they are reused to form new vesicles (endocytosis), filled with ACh, and then transported to the active sites for release.

Nerve Terminal Action Potential
During the nerve action potential, Na + from outside the nerve flows down its electrochemical gradient across the cell membrane and the resulting depolarizing voltage opens voltage-gated Ca 2+ channels, which permit Ca 2+ to enter the nerve down its electrochemical gradient, triggering the release of ACh. Of the several subtypes of Ca 2+ channels, four voltage-gated Ca 2+ channels are important for spontaneous and evoked release of ACh: P/Q-, N-, L-, and R-type channels. P/Q type channels are found only in nerve terminals immediately adjacent to the active zones and are probably the major type responsible for the normal release of neurotransmitter. They are voltage-gated and open and close in response to changes in the membrane voltage caused by the nerve action potential.
During the nerve action potential, the Ca 2+ current persists until the channels inactivate and outward fluxes of K + from inside the nerve return the membrane potential to normal. As with Ca 2+ channels on the nerve terminal, there are several forms of K + channels, including voltage-gated and Ca 2+ -activated potassium ion (K + ) channels. These K + channels limit the duration of nerve terminal depolarization and thereby Ca 2+ entry and release of neurotransmitter.
In the absence of an action potential, small spontaneous depolarizing potentials can be recorded at the postsynaptic muscle membrane. These miniature endplate potentials (MEPPs) are the result of the spontaneous release of a small number of ACh-containing vesicles. They have only 1% of the amplitude of the evoked potential produced when the motor nerve is stimulated. Statistical analysis has led to the conclusion that there is a minimum size for MEPPs and that the size of all MEPPs is equal to or a multiple of this minimum size, equivalent to the fusion of a single synaptic vesicle. Consequently, it was deduced that MEPPs are produced by these uniform sized packages or quanta of released transmitter in the absence of stimulation. Stimulation-evoked endplate potential depolarization is the additive effect produced by several hundred vesicles synchronously discharging quanta of neurotransmitter. Except for the reduced amplitude, MEPPs resemble the endplate potential in their time course and their sensitivity to drugs.
Acetylcholinesterase
Acetylcholinesterase is a type-B carboxylesterase enzyme located primarily in the synaptic cleft with a smaller concentration in the extrajunctional area. Acetylcholinesterase is secreted by the muscle and remains attached to it by collagen fastened to the basal lamina. ACh molecules that do not bind immediately with a receptor or those released after reacting with a receptor are hydrolyzed almost instantly (in less than 1 msec) by acetylcholinesterase. Approximately 50% of the released ACh is hydrolyzed into choline and acetate before reaching the receptor. Choline is taken up by the nerve terminal and reused for synthesis of ACh.
Acetylcholine Receptors
Structure
Nicotinic AChRs belong to the cys-loop superfamily of pentameric ligand-gated ion channels that share a common architecture, with five subunits surrounding a central ion-conducting pore. Each subunit is built on four transmembrane segments with the second transmembrane segment lining the pore ( Fig. 21.3 ). Each subunit is composed of approximately 400 to 500 amino acids. The AChRs are synthesized in muscle cells and anchored to the endplate membrane by the 43-kDa protein rapsyn. Four subtypes of perijunctional AChRs are of clinical importance: the presynaptic α 3 β 2 , the postsynaptic 2α 1 β 1 δε or 2α 1 β 1 δγ and the α 7 AChRs located both in terminal Schwann cell and postsynaptically on the muscle ( Fig. 21.4 ). The α subunits contain two adjacent cysteines essential for ACh binding and the non-α subunits contribute to the specificity and stability of each receptor isoform.


Neuronal AChRs include homomeric and heteromeric isoforms, with α 7-9 subunits forming homomeric (formed of same subunit) AChRs and heteromeric AChRs formed by a combination of α 2-6 and β 2-4 subunits with a stoichiometry of 2α and 3β. Compared with muscle AChRs, which have two distinct agonist binding sites—one high-affinity site between α 1 and δ and a lower-affinity site between α 1 and δ—neuronal AChR agonist binding sites vary based on the subunit composition. This variation contributes to the pharmacologic specificity of each receptor isoform. Heteromeric neuronal AChRs have two α-subunit binding sites between the α and β subunits, while the homomeric isoforms (e.g., α 7 ) have five potential binding sites, although the number of bound agonists required for receptor activation is not known.
Although many potential receptors are possible by combining different subunits, only a few have been found to be of biologic importance. In the NMJ, the α 3 β2 (neuronal) subtype is found in the presynaptic nerve ending. The 2α 1 β 1 δγ and α 7 AChRs are found postsynaptically during development and denervation and in other acquired pathologic states (e.g., immobilization and burns). NMBs and their metabolites can block ACh-mediated effects on neuronal AChRs in vitro. The α 7 AChRs are also constitutively expressed in terminal Schwann cells. Small amounts of NMBs do cross the blood-brain barrier, but larger amounts most likely cross in critical illness when the blood-brain barrier is more permeable. The long-term effects of NMBs on AChRs in the central nervous system are unclear, although inadvertent acute direct administration is known to cause seizures. Interestingly, NMBs decrease hypoxic ventilatory response in partially paralyzed humans; the mechanism for this response might be inhibition of neuronal AChRs within the carotid body.
Electrophysiology
The electrophysiologic technique of patch clamping has provided valuable insight into the functioning of prejunctional and postjunctional AChRs. With patch clamping, a glass micropipette is pressed onto the lipid membrane to form a seal. The pipette is filled with an ionic solution and conducts electric current to an amplifier. Depending on the configuration of the patch, the composition of the pipette or external solution can be changed to include drugs such as ACh, an NMB, or other drugs to study the ion channels under different conditions and the current or voltage monitored. Using these methods it is possible to determine how alterations in subunit composition, environment, or drugs change receptor electrophysiology.
Under normal conditions, the ion-conducting pore of the AChR is closed by the approximation of the pore gate. When an agonist such as ACh occupies both binding sites, a conformational change occurs, opening a channel through which ions flow along a concentration gradient. Both α subunits must be occupied simultaneously by agonist; if only one is occupied, the channel remains closed. The NMB binds to one or more of the α subunits, thus preventing ACh from binding and opening the ion channel. This interaction between ACh and NMB is competitive and the outcome—neuromuscular transmission or block—depends on the relative concentration and binding characteristics of the agonist (ACh) and antagonist (NMB).
The AChR ion channel accommodates many cations and electrically neutral molecules but excludes anions. The resulting depolarizing current measured by patch clamp recording through each open channel is minuscule (only a few picoamperes). However, each nerve terminal action potential releases enough ACh to open about 500,000 channels simultaneously, and the total current is more than adequate to depolarize the endplate and produce muscle contraction. This again is part of the increased margin of safety or reserve capacity of neurotransmission.
In addition to open or closed states, receptor channels are capable of a variety of other conformational current-passing states. They alter the amount of time that they remain open, open and close more slowly than usual, open briefly and repeatedly, or allow passage of fewer or more ions than they usually do (conductance). Receptor channels are dynamic structures that are influenced by a variety of drugs and changes in external milieu, including membrane fluidity, temperature, electrolyte balance, and chemical and physical factors. The net effect of these channel influences is ultimately reflected in the strength of neuromuscular transmission and muscle contraction.
Receptor Types
Postsynaptic Receptors
The trophic function of the nerve and the associated electrical activity are vital for the development, maturation, and maintenance of the NMJ. During the late embryonic stage, the motor nerve axons grow into the developing muscle and release growth factors, including agrin and neuregulins (NRβ 1 and NRβ 2 ) essential to the maturation of myotubules to muscle. Agrin, a nerve- and muscle-derived protein, stimulates postsynaptic differentiation by activating on a muscle-specific tyrosine kinase (MuSK). Agrin, together with neuregulins and other trophic factors, induces the clustering of AChRs and other essential muscle-derived proteins. including MuSK, rapsyn, and Erbβ, all of which are necessary for maturation and stabilization of AChRs at the NMJ.
During development (fetus), the postsynaptic AChRs are composed mainly of 2α 1 β 1 δγ and α 7 AChRs and spread throughout the muscle membrane (see Fig. 21.5 ). Just before birth and shortly thereafter, the 2α 1 β 1 δγ AChRs (also called immature AChRs ) are replaced by mature receptors with a subunit composition of 2α 1 β 1 δε and the receptors are clustered around the nerve. The immature receptors expressed in the fetus are more sensitive to depolarization by ACh, and this increased sensitivity might play a role in directing the nerve bud to muscle. The mechanism of the change from immature to mature receptors is unknown; however, the neuregulin NRβ 1 (also called ARIA ), which binds to one of the Erbβ receptors, seems to play a role. In the rodent, it takes about 2 weeks for complete conversion of immature to mature AChRs, whereas in humans this process takes longer. The mechanism and time course for disappearance of α 7 AChRs in muscle of the newborn in humans is unknown.
Presynaptic Receptors
Compared with postsynaptic AChRs, the structure and function of presynaptic AChRs are not as well understood. Differences exist between presynaptic and postsynaptic AChRs in their ability to bind toxins, as well as agonists and antagonists. Presynaptic AChRs are autoreceptors responsible for modulating the release of ACh into the synaptic cleft by means of a positive or negative feedback system. The positive feedback system is further regulated by a negative feedback system that senses when the concentration of transmitter in the synaptic cleft has increased to appropriate levels and shuts down further release. Targeted inhibition of presynaptic α 3 β 2 receptors during high-frequency repetitive stimulation results in typical tetanic fade (i.e., the decrease of twitch height during repetitive nerve-stimulated muscle contraction). This tetanic fade during experimental conditions equates to the train-of-four (TOF) fade seen clinically during neuromuscular monitoring after nondepolarizing NMBs and is used as an indicator of the presence of relaxant-induced residual muscle weakness (see Chapter 22 ). In contrast to block of presynaptic α 3 β 2 receptors produced by clinically used nondepolarizing NMBs, the depolarizing agent succinylcholine (SCh) does not inhibit α 3 β 2 receptors. This explains why TOF fade is absent during SCh-induced block. Recently the terminal Schwann cells have been shown to constitutively express α 7 AChRs. By selective inhibition of the acetylcholine esterase enzymes and α 7 AChRs and the use of α 7 AChRs knockout mice, the authors have demonstrated that α 7 AChRs expressed at the terminal Schwann cells act as sensors for spillover of ACh during neurotransmission. During excessive release of ACh, there is a feedback mechanism via the α 7 AChRs to inhibit the further release of ACh. These new findings on the role of α 7 AChRs in terminal Schwann cell in the control of ACh release needs further study and confirmation. Fade, however, is not always a prejunctional phenomenon, exemplified by the disease myasthenia gravis, a postjunctional disease that shows fade during repetitive stimulation. Fade in myasthenia gravis is due to decrease of postjunctional AChRs, reflecting decreased margin of safety. Thus from a practical point of view, fade observed during repetitive nerve stimulation reflects decreased margin of safety rather than a prejunctional or postjunctional phenomenon.
Mature and Immature Postjunctional Receptors
Mature postjunctional AChRs are composed of five subunits consisting of two α 1 subunits and one each of the β 1 , δ, and ε subunits (see Fig. 21.4 ). Although the innervated NMJ only synthesizes the mature form of AChR, expression of other receptor isoforms can be induced with decreased neural influence or activity, as seen, for example, in the fetus before innervation or in adult patients after denervation such as occurs with upper or lower motor neuron injury or after burn injury and immobilization. In these conditions, immature receptors consisting of 2α 1 β 1 δγ AChRs together with α 7 receptors are reexpressed ( Fig. 21.5 ). The immature heteromeric AChR is structurally similar to the mature AChR but with a γ subunit replacing the ε subunit. The immature receptors are also referred to as extrajunctional because they are expressed mostly, but not exclusively, in the extrajunctional region of the muscle membrane. The α 7 AChRs are homomeric composed of five α 7 subunits. Expression of α 7 AChRs has been demonstrated in fetal and denervated muscle and seems to be reexpressed in conditions such as immobilization, systemic inflammation, and burn injury and possibly in other pathologic states in which muscle wasting occurs (see later text).
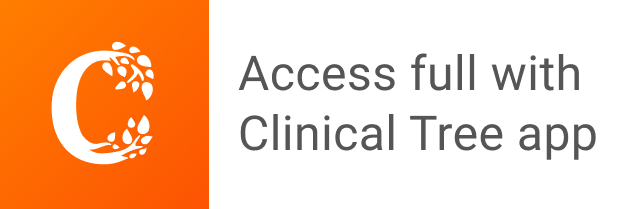