Key Points
- ▪
Good evidence-based practice dictates that clinicians always quantitate the extent of neuromuscular block by objective monitoring.
- ▪
The neuromuscular block should be adjusted to ensure optimal surgical conditions. In most procedures, one or two responses to train-of-four (TOF) stimulation will suffice. To avoid involuntary diaphragmatic movements, a deeper level of neuromuscular block is required (i.e., one to five responses to post-tetanic count [PTC]).
- ▪
Adequate recovery of postoperative neuromuscular function cannot be guaranteed without objective neuromuscular monitoring.
- ▪
Objective neuromuscular monitoring is essential for management of neuromuscular blockade intraoperatively and its reversal for postoperative care. Muscle relaxants should not be given in the intensive care unit without proper monitoring.
- ▪
It is impossible to exclude with certainty clinically significant residual neuromuscular block by clinical evaluation of recovery of neuromuscular function.
- ▪
Residual postoperative neuromuscular block causes decreased chemoreceptor sensitivity to hypoxia, functional impairment of the pharyngeal and upper esophageal muscles, impaired ability to maintain an open upper airway, and an increased risk of hypoxemic events, as well as the development of postoperative pulmonary complications.
- ▪
Absence of tactile fade in the response to TOF stimulation, tetanic stimulation, and double-burst stimulation does not exclude significant residual block.
- ▪
To exclude clinically significant residual neuromuscular block, the TOF ratio must exceed 0.9 when measured mechanically or electromyographically and 1.0 when measured acceleromyographically.
- ▪
Antagonism of the neuromuscular block with a cholinesterase inhibitor should not be initiated before at least two to four responses to TOF stimulation are observed.
- ▪
Antagonism of the neuromuscular block achieved by rocuronium and vecuronium can be initiated at all levels of block with the selective relaxant binding agent sugammadex.
- ▪
If adequate recovery (TOF ≥0.9-1.0) has not been documented objectively at the end of the surgical procedure, the neuromuscular block should be antagonized.
Acknowledgment
The editors, publisher, and Dr. Casper Claudius would like to thank Dr. Jorgen Viby-Mogensen for his contribution to this chapter in the prior edition of this work. It has served as the foundation of the current chapter.
Our understanding of the pathophysiologic consequences of residual paralysis has improved over the last decades, and it is now generally accepted that even small degrees of residual paralysis (i.e., a train-of-four [TOF] ratio 0.7-0.9) may be clinically harmful. As a consequence, the benchmark of adequate neuromuscular recovery has been revised several times; an adductor pollicis TOF ratio of 0.9 or greater is now required to exclude relevant residual neuromuscular block (i.e., paralysis). Clinically significant residual paralysis cannot be excluded using clinical criteria and it can persist postoperatively. Objective monitoring of the degree of neuromuscular block associated with pharmacologic reversal reduces the incidence of residual paralysis and should be part of standard perioperative monitoring when neuromuscular blocking agents (NMBAs) are used.
In awake patients, muscle power can be evaluated by tests of voluntary muscle strength, but this is impossible during anesthesia and recovery from anesthesia. Historically, anesthesiologists have used clinical tests to assess muscle power directly and to estimate neuromuscular function indirectly (muscle tone; feel of the anesthesia bag as an indirect measure of pulmonary compliance, tidal volume, and inspiratory force). All these tests are influenced by factors other than the degree of neuromuscular block and, therefore, should not be used to evaluate recovery from neuromuscular blockade. Whenever precise information regarding the status of neuromuscular functioning is desired, the response of muscle to nerve stimulation should be assessed. This procedure also takes into account the considerable variation in individual response and sensitivity to muscle relaxants.
This chapter reviews the basic principles of neuromuscular monitoring and the requirements for effective use of nerve stimulators for peripheral nerve stimulation. It also describes the response to nerve stimulation during depolarizing (phase I and phase II) and nondepolarizing neuromuscular block, provides information about the level of neuromuscular blockade, and discusses the consequences of residual paralysis. Moreover, methods of evaluating evoked neuromuscular responses with and without the availability of recording equipment are discussed.
Principles of Peripheral Nerve Stimulation
Neuromuscular monitoring is used to evaluate the effect of a NMBA. The muscle response after stimulation of its corresponding motor nerve is assessed. The most frequently assessed nerve-muscle unit is the ulnar nerve and the adductor pollicis muscle. The muscle response can be evaluated either qualitatively with a peripheral nerve stimulator or quantified with objective monitors. With the peripheral nerve stimulator, the observer evaluates the muscle response either tactically or visually, whereas with the monitor the response is objectively measured and displayed on a screen. Whatever method is used for neuromuscular monitoring, the clinician should be familiar with the following terms: supramaximal stimulation, calibration, impedance, and safety margin.
Supramaximal Stimulation
The reaction of a single muscle fiber to a stimulus follows an all-or-none pattern. In contrast, the response (the force of contraction) of the whole muscle depends on the number of muscle fibers activated. If a nerve is stimulated with sufficient intensity, all fibers supplied by the nerve will react, and the maximum response will be triggered. After administration of a neuromuscular blocking drug, the response of the muscle decreases in parallel with the number of fibers blocked. The reduction in response during constant stimulation reflects the degree of neuromuscular block.
For the preceding principles to work, the stimulus must be truly maximal throughout the whole period of monitoring; therefore, the electrical stimulus applied is usually at least 15% to 20% greater than that necessary for a maximal response. For this reason, the stimulus is said to be supramaximal. This compensates for potential changes in skin resistance intraoperatively and assures constant maximal stimulation throughout the procedure.
However, supramaximal electrical stimulation can be painful, which is not a concern during anesthesia, but during recovery the patient may be awake enough to experience the discomfort of nerve stimulation. Therefore, some researchers advocate stimulation with submaximal current during recovery. Although several investigations indicate that testing of neuromuscular function can be reliably performed postoperatively with submaximal stimulation, the accuracy of such monitoring is unacceptable with that low current.
Calibration
A device used for objective monitoring of the neuromuscular function should be calibrated before the NMBA is administered. Calibration adjusts the gain of the device to ensure that the observed response to supramaximal stimulation is within the measurement window of the device and as close as possible to the “100% control response.” The calibration procedure varies with the type of device used, but most often it is done with 1.0 Hz single-twitch stimulation. It is especially important to calibrate when the onset and recovery of the neuromuscular block are established with single-twitch stimulation.
In the TOF mode of nerve stimulation, calibration is considered less important because all four responses are amplified equally. Consequently, the TOF ratio is rarely influenced by calibration; however, in patients with very weak or strong responses to nerve stimulation, one or more responses to TOF stimulation might be out of the recording window, and the displayed TOF response might be incorrect. In some devices, supramaximal stimulation is established concurrently with the calibration procedure.
Impedance
An alternative and novel option to ensure a constant maximum stimulus throughout the whole procedure is to control the impedance (resistance) of the skin. Indeed, as long as the resistance of the skin is below a threshold value, the neuromuscular monitoring device will stimulate with the same user-selected electrical current (i.e., 60 mA). For a maximum current of 60 mA, the maximal resistance of the skin should be equal to or lower than 5 kΩ. If the resistance of the skin is above this value, the monitor will not be able to stimulate the patient with the selected current. More recently, nerve stimulators have been introduced that indicate the level of skin impedance on the screen (e.g., TofScan by iDMed, Marseille, France). Using this approach, establishment of supramaximal stimulation is not needed to assure that nerve stimulation is effective and constantly maximal through the whole procedure.
Safety Margin
Neuromuscular transmission has a substantial margin of safety. Neuromuscular block only becomes evident when 70% to 80% of acetylcholine receptors at the neuromuscular endplate are occupied by nondepolarizing NMBDs and to produce complete block, 90% to 95% of receptors must be occupied. Thus, the currently available equipment and the currently applied stimulation patterns allow only insight to this 70% to 95% range of receptor occupancy. This should be kept in mind, especially during recovery of neuromuscular block, where 70% of the acetylcholine receptors at the neuromuscular endplate may still be occupied but no longer detectable with neuromuscular monitoring.
Types of Peripheral Nerve Stimulation
Neuromuscular function is monitored by evaluating the muscular response to supramaximal stimulation of a peripheral motor nerve. Theoretically, two types of stimulation can be used: electrical and magnetic. Electrical nerve stimulation is by far the most commonly used method in clinical practice, and it is described in detail in this chapter. In theory, magnetic nerve stimulation has several advantages over electrical nerve stimulation. It is less painful and does not require physical contact with the body; however, the equipment required is bulky and heavy, it cannot be used for TOF stimulation, and it is difficult to achieve supramaximal stimulation with this method. As a result, magnetic nerve stimulation is not used in clinical anesthesia.
Basic Considerations
Stimulating Electrodes
Electrical impulses are transmitted from stimulator to nerve by means of surface or needle electrodes. Normally, disposable pre-gelled silver or silver chloride surface electrodes are used. The conducting area should be small, approximately 7 to 11 mm in diameter ( Fig.43.1 ). Otherwise, the current produced in the underlying nerve may not be adequate. Ideally, the skin should be cleansed properly and preferably rubbed with an abrasive before application of the electrodes. When the selected current cannot be obtained with surface electrodes, needle electrodes can be used in a few exceptional cases. Although specially coated needle electrodes are commercially available, ordinary steel injection needles often suffice. A sterile technique should be used, and the needles should be placed subcutaneously to avoid direct injury to the underlying nerve.
Sites of Nerve Stimulation and Different Muscle Responses
In principle, any superficially located peripheral motor nerve can be stimulated and the response to corresponding muscle measured. Choosing the site of neuromuscular monitoring depends on several factors: the site should be easily accessible during surgery, it should allow quantitative monitoring and finally, direct muscle stimulation should be avoided. Direct muscle stimulation is characterized by weak contractions without fade persisting even at a deep level of neuromuscular blockade. The risk is increased when the stimulation electrodes are directly attached over the muscle to be assessed. To prevent direct muscle stimulation, the nerve-muscle unit should be chosen so that the site of nerve stimulation and the site of the subsequent evaluation of the twitch response are topographically (anatomically) distinct.
In clinical anesthesia, the ulnar nerve is the gold standard as a stimulation site, but the median, posterior tibial, common peroneal, and facial nerves are also sometimes used. For stimulation of the ulnar nerve, the electrodes are best applied to the volar side of the wrist (see Fig. 43.1 ). The distal electrode should be placed approximately 1 cm proximal to the point at which the proximal flexion crease of the wrist crosses the radial side of the tendon to the flexor carpi ulnaris muscle. The proximal electrode should preferably be placed so that the distance between the centers of the two electrodes is 3 to 6 cm (see Fig. 43.1 ). With this placement of the electrodes, electrical stimulation normally elicits only finger flexion and thumb adduction. If one electrode is placed over the ulnar groove at the elbow, thumb adduction is often pronounced because of stimulation of the flexor carpi ulnaris muscle. When this latter placement of electrodes (sometimes preferred in small children) is used, the active negative electrode should be at the wrist to ensure maximal response. Polarity of the electrodes is less crucial when both electrodes are close to each other at the volar side of the wrist; however, placement of the negative electrode distally normally elicits the greatest neuromuscular response. When the temporal branch of the facial nerve is stimulated, the negative electrode should be placed over the nerve, and the positive electrode should be placed somewhere else over the forehead. When the posterior tibial nerve is stimulated, the electrodes should be placed close to the medial malleolus, with the same distance as described above and the negative electrode being placed distally.

Nerve-Muscle Unit
Several nerve-muscle units may be chosen in clinical practice. Most often the ulnar nerve-adductor pollicis muscle is used.
Ulnar nerve-adductor pollicis muscle: This nerve-muscle unit is easily accessible intraoperatively if the arm is in the outstretched position and the hand in the supine position. The stimulatory response can be evaluated tactilely, visually, or by objective means. It has the lowest risk of direct muscle stimulation, because it ensures topographic separation of the stimulated nerve and the evaluated muscle by stimulating the ulnar nerve running along the median side of the arm and assessing the muscle response at the adductor pollicis muscle, which is indeed located on the lateral side of the hand.
Posterior tibial nerve-flexor hallucis brevis muscle: This nerve-muscle unit can be used for monitoring when the hands are inaccessible. The flexor hallucis brevis muscle produces flexion of the big toe following posterior tibial nerve stimulation. The characteristics (onset and recovery) of the neuromuscular block at the flexor hallucis brevis muscle is almost consistent with that of the adductor pollicis muscle.
Facial nerve-orbicularis oculi and facial nerve-corrugator supercilii muscle: When the arms are tucked under surgical drapes, quite often the only accessible site for monitoring is the head. Two facial muscles can be used as monitoring sites: the orbicularis oculi muscle and the corrugator supercilii muscle. The former encircles the orbital opening; its stimulation through the zygomatic branches of the facial nerve causes the eyelids to close. Stimulation by the temporal branch of the facial nerve of the latter one draws the medial end of the eyebrow downward, producing wrinkling of the brow. However, because the facial nerve is in direct proximity to the intrinsic mimic muscles, the risk of direct muscle stimulation is significant. Therefore, care must be taken that the correct stimulatory response is assessed, and any other twitching muscle in the direct proximity of the stimulation electrodes is not falsely interpreted. Stimulation of the facial nerve can be accomplished with significantly lower currents: most often 25 to 30 mA are sufficient. Stimulation of these two muscles is technically difficult and the result often unsatisfactory in clinical practice.
Because different muscle groups have different sensitivities to neuromuscular blocking drugs, results obtained for one muscle cannot be automatically extrapolated to other muscles. However, most of the studies that are the base for dosing recommendations of NMBAs arise from measurement of the stimulation of the ulnar nerve. The diaphragm is among the most resistant of all muscles to both depolarizing and nondepolarizing neuromuscular blocking drugs. In general, the diaphragm requires 1.4- to 2.0-fold as much muscle relaxant as the adductor pollicis muscle for an identical degree of block ( Fig. 43.2 ). Also of clinical significance is that onset time is normally shorter for the diaphragm than for the adductor pollicis muscle, and the diaphragm recovers from paralysis more quickly than the peripheral muscles ( Fig. 43.3 ). The other respiratory muscles are less resistant than the diaphragm, as are the larynx and the corrugator supercilii muscles. Most sensitive are the abdominal muscles, the orbicularis oculi muscle, the peripheral muscles of the limbs, and the geniohyoid, masseter, and upper airway muscles. From a clinical point of view, the response of the corrugator supercilii to facial nerve stimulation reflects the extent of neuromuscular block of the laryngeal adductor muscles and abdominal muscles better than the response of the adductor pollicis to ulnar nerve stimulation. Furthermore, the upper airway muscles seem to be more sensitive than the peripheral muscles. Although some investigations using acceleromyography (AMG) have indicated small differences in the response to TOF nerve stimulation in the hand (adductor pollicis muscle) compared to the leg (flexor hallucis brevis muscle), these differences are probably of little clinical significance. When comparing different sites of stimulation, there might be large differences between contralateral limbs (e.g., arm-to-arm variation of ± 20%).


Although the precise source of these differences is unknown, possible explanations may be variations in acetylcholine receptor density, acetylcholine release, acetylcholinesterase activity, fiber composition, innervation ratio (number of neuromuscular junctions), blood flow, and muscle temperature.
Patterns of Nerve Stimulation
For the evaluation of the neuromuscular function, the most commonly used patterns are TOF stimulation, double-burst stimulation (DBS), and posttetanic count (PTC) stimulation. Single-twitch stimulation and tetanic stimulation are mainly used as a component in composite stimulation patterns (i.e., TOF, DBS, or PTC).
Single-Twitch Stimulation
Background : Single-twitch stimulation is the earliest and simplest pattern. The first device specifically developed to monitor the neuromuscular block, the “St. Thomas’s Hospital nerve stimulator,” could only deliver a single twitch. For decades it remained the only established stimulation pattern to assess neuromuscular blockade intraoperatively.
Stimulation pattern : In the single-twitch mode of stimulation, single electrical stimuli are applied to a peripheral motor nerve at frequencies ranging from 1.0 Hz (once every second) to 0.1 Hz (once every 10 seconds; Fig. 43.4 ) and the subsequent muscle response is evaluated. The response to single-twitch stimulation depends on the frequency at which the individual stimuli are applied. If the rate of delivery is increased to greater than 0.15 Hz, the evoked response will gradually decrease and stabilize at a lower level. Therefore, results obtained with 1-Hz single-twitch stimulation cannot be compared with results obtained using, for example, 0.1-Hz single-twitch stimulation. As a result, a frequency of 0.1 Hz is generally recommended.

Clinical application : To assess the degree of neuromuscular blockade after single-twitch stimulations, a comparison with a reference value recorded before administration of the NMBA is mandatory. Thus, without appropriate monitoring equipment, this stimulation pattern does not provide sufficient information of the level of block. In clinical practice, the single twitch stimulation has only limited value as a stand-alone stimulation pattern; it is mainly used as a component of the PTC stimulation and as 0.1 Hz single-twitch stimulation, it is sometimes used in scientific trials specifically to evaluate the time to onset of neuromuscular blockade. Moreover, it is the only stimulation pattern that allows, in conjunction with a monitoring device, assessing a depolarizing neuromuscular block after succinylcholine.
Train-of-Four Stimulation
Background: The TOF stimulation pattern was introduced by Ali and associates during the early 1970s. They aimed to develop a tool providing clinically reliable information throughout all phases of neuromuscular blockade with simple nerve stimulator and without the need for a monitoring device.
Stimulation pattern : TOF stimulation consists of four supramaximal stimuli given every 0.5 seconds (2 Hz; Fig.43.5 ) and each stimulus in the train causes the muscle to contract. The basis for evaluation is either the number of discernible responses after TOF stimulation (i.e., TOF count) or the “fade” in the train of responses—that is, dividing the amplitude of the fourth response by the amplitude of the first response (i.e., TOF ratio). Without prior administration of a muscle relaxant, all four responses are normally the same: the TOF ratio is 1.0. During a partial nondepolarizing block, the TOF ratio decreases (fades) and is inversely proportional to the degree of block. During a partial depolarizing block, no fade occurs in the TOF response and the TOF ratio remains 1.0, independently of the level of depolarizing neuromuscular blockade. Fade in the TOF response after injection of succinylcholine signifies the development of a phase II block (discussed later in the section on depolarizing neuromuscular block).

When used continuously, an interval of at least 10 seconds should be allowed between each set (train) of four stimuli to avoid fade during the measurement.
Application: TOF stimulation is still the most frequently used stimulation pattern. The advantages of TOF stimulation are most apparent during a nondepolarizing neuromuscular block because the degree of block can be read directly from the TOF response even though a preoperative value is lacking. Clinically relevant information about the onset, surgical relaxation, and neuromuscular recovery can be obtained with the same stimulation pattern by using a simple peripheral nerve stimulator; the TOF count allows reliable assessment of the onset of neuromuscular block, and moderate blockade. Moreover, the TOF ratio can be taken as a measure of neuromuscular recovery from nondepolarizing blockade. TOF stimulation has some advantages over DBS and PTC stimulation; it is less painful and, unlike tetanic stimulation, does not generally influence subsequent monitoring of the degree of neuromuscular block. There are major limitations of the TOF stimulation pattern. First, the subjective assessment of the TOF ratio overestimates neuromuscular recovery, as the tactile of visual estimation of fade is accurate only if the TOF ratio is less than 0.4; in other words, at a TOF ratio between 0.4 and 0.9, fade cannot be detected either visually or tactically. Therefore, objective monitoring devices are needed to further quantify neuromuscular recovery and to reliably exclude residual paralysis. Second, the TOF stimulation does not allow the clinician to quantify intense and deep levels of neuromuscular blockade (i.e., at no responses to TOF). Finally, the TOF stimulation does not allow monitoring of the depolarizing phase I blockade.
Double-Burst Stimulation
Background : DBS was developed by Viby-Mogensen and associates in 1989 to improve the tactile or visual evaluation of recovery from nondepolarizing neuromuscular blockade.
Stimulation pattern : DBS consists of two short bursts of 50-Hz tetanic stimulation separated by 750 ms, with a 0.2-ms duration of each square wave impulse in the burst ( Fig. 43.6 ). The number of impulses in each burst can vary: in the DBS 3,3 mode, there are three impulses in each of the two bursts, whereas in the DBS 3,2 mode, the first burst had three impulses and the second burst consisted of only two impulses. The individual twitches elicited by each burst blend together and are felt as one single muscle contraction. Thus, the response to DBS is two short muscle contractions and fade in the second burst compared to the first one is the basis for evaluation. In nonparalyzed muscle, both muscle contractions are of equal strength. In a partially paralyzed muscle, the second response is weaker than the first and corresponds to the typical TOF fade (see Fig 43.6 ). When measured mechanically, the TOF ratio correlates closely with the DBS 3,3 ratio. Compared to DBS 3,3, tactile detection of fade is slightly improved with the DBS 3,2 mode, especially at higher TOF ratios.

Clinical application : DBS was developed with the specific aim of improving manual (tactile or visual) detection of residual nondepolarizing block under clinical conditions, or during recovery and immediately after surgery. Indeed, tactile evaluation of the response to DBS is more accurate in detecting fade compared to TOF. However, a DBS is still insufficient to exclude reliably residual paralysis corresponding to a TOF ratio between 0.6 and 0.9. Accordingly, DBS cannot replace objective monitoring.
Tetanic Stimulation
Background : Tetanic stimulation was proposed by Tassonyi in 1975 as an alternative method to evaluate residual neuromuscular blockade.
Stimulation pattern : Tetanic stimulation consists of high-frequency delivery of electrical stimuli (e.g., 50-100 Hz). The most commonly used pattern in clinical practice is 50-Hz stimulation given for 5 seconds, although some investigators have advocated the use of 100-Hz, or even 200-Hz stimulation for 1 second. In normal neuromuscular transmission, the observer detects one strong, sustained muscle contraction, and fade after tetanic stimulation is the basis for evaluation of nondepolarizing block. During a depolarizing block, the muscle response to 50-Hz tetanic stimulation for 5 seconds is sustained. In contrast, during a phase II block after the injection of succinylcholine, the response following tetanic stimulation is not sustained (i.e., fade occurs; Fig. 43.7 ).

Fade in response to tetanic stimulation is normally considered a presynaptic event. The traditional explanation is that at the start of tetanic stimulation, large amounts of acetylcholine are released from immediately available stores in the presynaptic nerve terminal. As these stores become depleted, the rate of acetylcholine release decreases until equilibrium between mobilization and synthesis of acetylcholine is achieved. Despite this equilibrium, the muscle response to tetanic nerve stimulation is maintained (given normal neuromuscular transmission) because the acetylcholine released is many times greater than the amount necessary to evoke a response. However, when the “margin of safety” at the postsynaptic membrane (i.e., the number of free cholinergic receptors) is reduced by nondepolarizing neuromuscular blocking drugs, a typical reduction in twitch height is seen with a fade, especially during repetitive stimulation. In addition to this postsynaptic block, nondepolarizing neuromuscular blocking drugs can also block presynaptic neuronal subtype acetylcholine receptors, thereby leading to impaired mobilization of acetylcholine within the nerve terminal. This effect substantially contributes to fade in the response to tetanic (and TOF) stimulation. Although the degree of fade depends primarily on the degree of neuromuscular block, fade also depends on the frequency (Hz), the length (seconds) of stimulation, and on how often tetanic stimuli are applied. Unless these variables are kept constant, results from different studies using tetanic stimulation cannot be compared.
Clinical application: Traditionally, tetanic stimulation was proposed to evaluate residual neuromuscular block. While the sensitivity of tetanic stimulation to detect residual paralysis is about 70%, its specificity is only about 50%. Especially when anesthesia was maintained with volatile anesthetics, a marked fade can be observed despite adequate neuromuscular recovery or even without prior administration of a nondepolarizing NMBA. Hence, this test is of limited value for assessing neuromuscular recovery. Furthermore, tetanic stimulation is very painful, which limits its use in unanesthetized patients. In the late phase of neuromuscular recovery, tetanic stimulation can produce lasting antagonism of neuromuscular block in the stimulated muscle such that the response of the tested site may no longer be representative of other muscle groups. For all these reasons, tetanic stimulation has little, if any, use in everyday clinical anesthesia, except as a component in DBS and PTC stimulation.
Posttetanic Count Stimulation
Background : PTC stimulation was developed by Viby-Mogensen to allow tactile or visual evaluation of profound nondepolarizing neuromuscular blockade that does not respond to TOF stimulation.
Stimulation pattern : PTC is a composite stimulation pattern composed by a tetanic stimulation (50 Hz for 5 seconds) followed by 10 to 15 single twitches (i.e., PTC twitches) given at 1 Hz starting 3 seconds after the end of tetanic stimulation. It is based on a phenomenon called “posttetanic potentiation”: tetanic stimulation leads to a transient, exaggerated release of acetylcholine that briefly shifts the ratio of acetylcholine and NMBA at the motor endplate in favor of acetylcholine. Even if no twitch response has been discernible prior to the tetanic stimulation, noticeable muscle contractions might occur briefly after tetanic stimulation (see Fig. 43.7 ). The basis for evaluation of the PTC is the count of these discernible posttetanic twitches.
The response to PTC stimulation depends primarily on the degree of neuromuscular block. It also depends on the frequency and duration of tetanic stimulation, the length of time between the end of tetanic stimulation and the first posttetanic stimulus, the frequency of the single-twitch stimulation, and probably the duration of single-twitch stimulation before tetanic stimulation. When the PTC method is used, these variables should be kept constant. Because of interference between PTC stimulation and the actual neuromuscular block within the monitored hand, tetanic stimulation should ideally not be performed more often than every 6 minutes.
Clinical application and limitation : Moderate levels of neuromuscular blockade (i.e., TOF count between 1 and 4) are not sufficient to reliably prevent reactions of the diaphragm and/or laryngeal muscles after stimulation. Both muscles are rather resistant to the effect of nondepolarizing NMBA. Therefore, more profound levels of neuromuscular blockade are needed in clinical situations where any bucking or coughing in response to tracheal stimulation or sudden diaphragmatic movements during surgery should be avoided. Only PTC stimulation allows assessment of these degrees of neuromuscular blockade. During intense block, there is no response to either tetanic or posttetanic stimulation ( Fig. 43.8 ). As the period of intense neuromuscular block dissipates, the first response to posttetanic twitch stimulation occurs and is followed by a gradual return of posttetanic twitches until the first response to TOF stimulation reappears. The PTC should be 3 or less if a deep block is required for clinical reasons; at 6 to 10 PTC, the return of the first TOF response is most often imminent ( Fig. 43.9 ). Because tetanic stimulation should not be performed more often than every 6 minutes, this stimulation pattern cannot be applied continuously.


Equipment
Although many nerve stimulators are commercially available, not all meet the basic requirements for clinical use. The stimulus should produce a monophasic and rectangular waveform, and the length of the pulse should not exceed 0.2 to 0.3 ms. A pulse exceeding 0.5 ms may stimulate the muscle directly or cause repetitive firing. Stimulation at a constant current is preferable to stimulation at a constant voltage because current is the determinant of nerve stimulation. Furthermore, for safety reasons, the nerve stimulator should be operated by a rechargeable battery, include a battery check, and be able to generate 60 to 70 mA, but not more than 80 mA. Some commercially available stimulators can deliver just 25 to 50 mA and provide a constant current only when skin resistance ranges from 0 to 2.5 kΩ. This is a limitation, as skin resistance can increase to approximately 5 kΩ, especially during lower skin temperature. The high skin resistance can cause the current delivered to the nerve to decrease below the supramaximal level and lead to a decrease in the response to stimulation. Ideally, the nerve stimulator should have a built-in warning system, or a current level display that alerts the user when the selected current is not delivered to the nerve. Alternatively, the impedance should be indicated at the screen. The polarity of the electrodes should be indicated, and the apparatus should be capable of delivering the following modes of stimulation: TOF (as both a single train and in a repetitive mode, with TOF stimulation being given every 10-20 seconds), and PTC. Some recent nerve stimulators switch automatically between TOF and PTC, depending on the level of neuromuscular blockade. If the nerve stimulator does not allow objective measurement of the response to TOF stimulation, at least one DBS mode should be available, preferably DBS 3,2 .
Peripheral Nerve Stimulator
Peripheral nerve stimulators only allow stimulation of the target nerve; the subsequent muscular response is assessed subjectively either tactilely or visually. When applying TOF stimulation and evaluating the TOF count, the peripheral nerve stimulator may deliver clinically useful information about the onset of neuromuscular block or the need for an additional dose of relaxant. Moreover, the clinician could be guided for the timing and dosing of reversal agents. It is in the determination of full neuromuscular recovery these devices have clinical limitations. It is impossible to reliably exclude residual paralysis. A simple nerve stimulator acts only as a guide and should not be used as a diagnostic tool to exclude residual paralysis.
Objective Monitors
Objective monitors measure the evoked responses objectively and display it on a screen.
Several methods may be used for objective clinical monitoring of neuromuscular function: evoked mechanical response of the muscle (mechanomyography [MMG]), evoked electrical response of the muscle (electromyography [EMG]), acceleration of the muscle response (AMG), evoked electrical response in a piezoelectric film sensor attached to the muscle (kinemyography [KMG]), measurement of pressure changes in blood pressure cuff after contractions of the upper arm muscles (cuff pressure modality [CPM]), measurement of pressure change of a spherical balloon after hand contraction (compressomyography [CMG]), and measurement of low-frequency sounds evoked by the muscle contraction (phonomyography [PMG]). The different methods are described below. For further information on recording evoked responses, the reader is referred to guidelines for good clinical research practice in pharmacodynamic studies of neuromuscular blocking drugs. The only objective monitors currently available are based on AMG, EMG, CPM, and KMG. The use of computer-guided administration of neuromuscular blocking drugs and “closed loop control” systems has been suggested, but no systems are commercially available.
Mechanomyography
MMG measures the isometric contraction of a muscle after stimulation of the corresponding nerve. A transducer converts the force of an isometric contraction into an electrical signal. For correct and reproducible measurement, the muscle contraction needs to be isometric. In clinical anesthesia, this condition is most easily achieved by measuring the force of contraction of the thumb after the application of a resting tension of 200 to 300 g (a preload) to the thumb. When the ulnar nerve is stimulated, the thumb (the adductor pollicis muscle) acts on a force-displacement transducer ( Fig. 43.10 ). The force of contraction is then converted into an electrical signal, which is amplified, displayed, and recorded. The arm and hand should be rigidly fixed, and care should be taken to prevent overloading of the transducer. In addition, the transducer should be placed in correct relation to the thumb (i.e., the thumb should always apply tension along the length of the transducer). It is important to remember that the response to nerve stimulation depends on the frequency with which the individual stimuli are applied and that the time used to achieve a stable control response may influence subsequent determination of the onset time and duration of block. Generally, the reaction to supramaximal stimulation increases during the first 8 to 12 minutes after commencement of the stimulation (staircase phenomenon). Therefore, in clinical studies, recording of the control response (before injection of muscle relaxant) should not be made until the response has stabilized for 8 to 12 minutes or a 2- or 5-second 50-Hz tetanic stimulation has been given. Even then, twitch response often recovers to 110% to 150% of the control response after paralysis with succinylcholine. This increase in response, possibly caused by a change in the contractile response of the muscle, normally disappears within 15 to 25 minutes.

Although there are numerous methods for mechanical recording of evoked mechanical responses, not all meet the criteria outlined. MMG is recognized as the gold standard of neuromuscular monitoring.
Despite this status, there is no commercially available neuromuscular monitor for daily clinical use based on this principle. This type of monitor is relegated to research purpose only.
Electromyography
EMG is the oldest technique used for quantification of neuromuscular blockade. Evoked EMG records the compound muscle action potentials produced by stimulation of a peripheral nerve. The compound action potential is an electrical activity that for many years could be detected only by means of a preamplifier and a storage oscilloscope. Modern neuromuscular transmission analyzers are able to make online electronic analyses and graphic presentations of the EMG response.
The evoked EMG response is most often obtained from muscles innervated by the ulnar or the median nerves. Stimulating electrodes are applied as in force measurements. Most often, the evoked EMG response is obtained from the thenar or hypothenar eminence of the hand or from the first dorsal interosseous muscle of the hand, preferably with the active electrode over the motor point of the muscle ( Fig. 43.11 ). The signal picked up by the analyzer is processed by an amplifier, a rectifier, and an electronic integrator. The results are displayed either as a percentage of control or as a TOF ratio.

Two new sites for recording the EMG response have been introduced: the larynx and the diaphragm. Using a noninvasive disposable laryngeal electrode attached to the tracheal tube and placed between the vocal cords, it is possible to monitor the onset of neuromuscular block in the laryngeal muscles. However, the method is mainly of interest in clinical research when investigating onset times of the laryngeal muscles. In paravertebral surface diaphragmatic EMG, the recording electrodes are placed on the right of vertebrae T12/L1 or L1/L2 for monitoring the response of the right diaphragmatic crux to transcutaneous stimulation of the right phrenic nerve at the neck. As is the case with surface laryngeal EMG, surface diaphragmatic EMG is mainly of interest in clinical research.
Evoked electrical and mechanical responses represent different physiologic events. Evoked EMG records changes in the electrical activity of one or more muscles, whereas evoked MMG records changes associated with excitation-contraction coupling and contraction of the muscle as well. For these reasons, the results obtained with these methods may differ. Although evoked EMG responses generally correlate well with evoked mechanical responses, marked differences can occur, especially in the response to succinylcholine and in the TOF ratio during recovery from a nondepolarizing block.
In theory, recording of evoked EMG responses has several advantages over recording of evoked mechanical responses. Equipment for measuring-evoked EMG responses is easier to set up, the response reflects only factors influencing neuromuscular transmission, and the response can be obtained from muscles not accessible to mechanical recording. However, evoked EMG does entail some difficulties. Although high-quality recordings are possible in most patients, the results are not always reliable. For one thing, improper placement of electrodes can result in inadequate pickup of the compound EMG signal. If the neuromuscular transmission analyzer does not allow observation of the actual waveform of the compound EMG, determining optimal placement of the electrodes is difficult. Another source of unreliable results may be that fixation of the hand with a preload on the thumb might be more important than is generally appreciated, inasmuch as changes in the position of the electrodes in relation to the muscle can affect the EMG response. In addition, direct muscle stimulation sometimes occurs. If muscles close to the stimulating electrodes are stimulated directly, the recording electrodes can pick up an electrical signal even though neuromuscular transmission is completely blocked. Another difficulty is that the EMG response often does not return to the control value. Whether this situation is the result of technical problems, inadequate fixation of the hand, or changes in temperature is unknown ( Fig. 43.12 ). Finally, the evoked EMG response is highly sensitive to electrical interference, such as that caused by diathermy.

Currently, to our knowledge, there are only a few EMG-based monitors available for clinical use, but more devices are under development.
Acceleromyography
AMG was developed specifically for clinical use and is widely practiced. The technique of AMG is based on Newton’s second law: force = mass × acceleration, it measures the isotonic acceleration of the stimulated muscle. If mass is constant, acceleration is directly proportional to force. Accordingly, after nerve stimulation, one can measure not only the evoked force but also acceleration of the thumb.
AMG uses a piezoelectric ceramic wafer with electrodes on both sides. Exposure of the electrode to a force generates an electrical voltage proportional to acceleration of the electrode. Consequently, when an accelerometer is fixed to the thumb and the ulnar nerve is stimulated, an electrical signal is produced whenever the thumb moves ( Fig. 43.13 ). This signal can be analyzed in a specially designed analyzer or displayed on a recording system.

AMG is a simple method of analyzing neuromuscular function, both in the operating room and in the intensive care unit. Although good correlation exists between the TOF ratio measured by this method and the TOF ratio measured with a force-displacement transducer or EMG, measurements made via AMG are not directly comparable with results obtained by the other two methods. When AMG is used with a free-moving thumb, as originally suggested, wide limits of agreements in twitch height (T1) and TOF ratio and differences in the onset and recovery course of block between AMG and MMG have been found. Moreover, the AMG control TOF ratio is consistently higher than when measured with a force-displacement transducer. In accordance with this, several studies have indicated that when using AMG, the TOF ratio indicative of sufficient postoperative neuromuscular recovery is 1.0 rather than 0.90, as when measured by MMG or EMG in the adductor pollicis muscle. In contrast to MMG and EMG, the control baseline TOF value before administration of a neuromuscular blocking drug is most often 1.1 to 1.2 when measured with AMG, and in some patients is as high as 1.4. A high control baseline value probably indicates that the TOF ratio necessary for excluding residual curarization is equally higher. For instance, in a patient with a high control baseline value (e.g., TOF = 1.2), it is to be expected that a higher TOF ratio during recovery is necessary to exclude residual block compared with a patient with a low control baseline value (e.g., TOF = 0.95). It is generally accepted that the TOF ratio should be at least 0.90 to exclude clinically significant residual paralysis; using the preceding example, a TOF ratio of 1.08 (90% of 1.2) would represent safe recovery in the first patient, whereas a TOF ratio of 0.86 (90% of 0.95) would suffice in the other patient. To overcome such problems, it has been suggested to refer the actually obtained TOF ratios during recovery to the baseline control TOF ratio (normalization). Currently, no commercially available monitors can “normalize” the TOF ratio automatically. Intuitively, for excluding residual block using AMG, a TOF ratio of at least 1.0 should be targeted to exclude residual block.
One reason for the wide limits of agreement between AMG and MMG is probably and paradoxically connected with one of the originally claimed advantages of the method, that fixation of the hand could be reduced to a minimum as long as the thumb could move freely. In clinical practice, it is often not possible to ensure that the thumb can move freely and that the position of the hand does not change during the surgical procedure. The evoked response can therefore vary considerably. Several solutions have been proposed, but the use of an elastic preload on the thumb improves the precision without compromising the agreement between results obtained with AMG and MMG ( Fig. 43.14 ). Several studies have indicated that objective monitoring with AMG reduces and almost eliminates the problem of postoperative residual neuromuscular block.

When the thumb is not available for monitoring during surgery, some clinicians prefer to monitor the AMG response of the orbicularis oculi or the corrugator supercilii in response to facial nerve stimulation. However, neuromuscular monitoring of both sites with AMG is subject to both large uncertainty regarding the extent of paralysis and high risk of direct muscle stimulation, and it cannot be recommended for routine monitoring. It provides only a rough estimate of the degree of block of the peripheral muscles.
AMG was one of the first widely distributed commercially available monitors and has therefore become the standard for qualitative monitoring in the clinical setting. Today, AMG devices are available as portable monitors as well as integrated in the anesthesia monitor.
Recently, AMG monitors with three-dimensional piezoelectric transducers were introduced, which sense the motion of the thumb in all directions, and not just in one plane. This might further improve the reliability of the AMG technology. This novel method has been compared to the TOF-Watch (one-dimensional AMG) in two small studies. Although both studies showed some disagreement between the two methods, the authors agreed that the three-dimensional monitor may be used in clinical practice. An advantage seems to be that the monitors’ integrated internal check-up ensuring that all components including the piezoelectric element are functional is developed. The newest monitors also display the impedance and calculate automatically a modified normalized TOF ratio. Hopefully, the new generation of AMG monitors becomes even more user-friendly and more reliable.
Kinemyography
The technique of KMG is based on the principle that stretching or bending a flexible piezoelectric film (e.g., one attached to the thumb) in response to nerve stimulation generates a voltage that is proportional to the amount of stretching or bending.
Few studies have evaluated the function of these monitors. Limited data indicate not only a good relationship between results obtained with KMG, AMG, and MMG, but also wide limits of agreement between the methods. Therefore, although KMG may be a valuable clinical tool, the values obtained in an individual patient with this method can vary from those obtained with MMG or AMG. Only one device based on this principle is available commercially in two sizes (adult and pediatric): the NMT MechanoSensor (Datex-Ohmeda, Helsinki, Finland).
Cuff Pressure Modality (CPM)
The cuff modality detects changes in cuff pressure due to muscle contraction. Electrodes integrated into a blood pressure cuff stimulate the brachial plexus at the humeral level. The subsequent bulk contraction of the upper arm generates pressure change in the blood pressure cuff which is analyzed and displayed at the monitor. However, only limited data are currently available and further clinical investigations are needed to prove the reliability and reproducibility of the new monitoring modality. One monitor based on this technology is commercially available: the TOF-Cuff NMT monitor (RGB Medical devices, Madrid, Spain).
Compressomyography
CMG measures pressure changes in a hand-held balloon. Following ulnar nerve stimulation, the force of muscle contraction of the hand muscles is transmitted to a balloon secured in the patient’s hand. Despite encouraging results of the only publication investigating the device, this technique has not been further developed and is not commercially available.
Phonomyography
PMG measures the intrinsic low-frequency sounds of muscle contraction with special microphones following nerve stimulation. PMG has been evaluated for clinical and research purposes by one study group. This group reports good correlation between evoked acoustic responses and those obtained with more traditional methods of recording, such as MMG, EMG, and AMG. A potential advantage of PMG, however, is that the method can be applied not only to the adductor pollicis muscle, but also to other muscles of clinical interest such as the diaphragm, larynx, and eye muscles. In addition, the ease of application is attractive. However, PMG-based monitors are not currently commercially available.
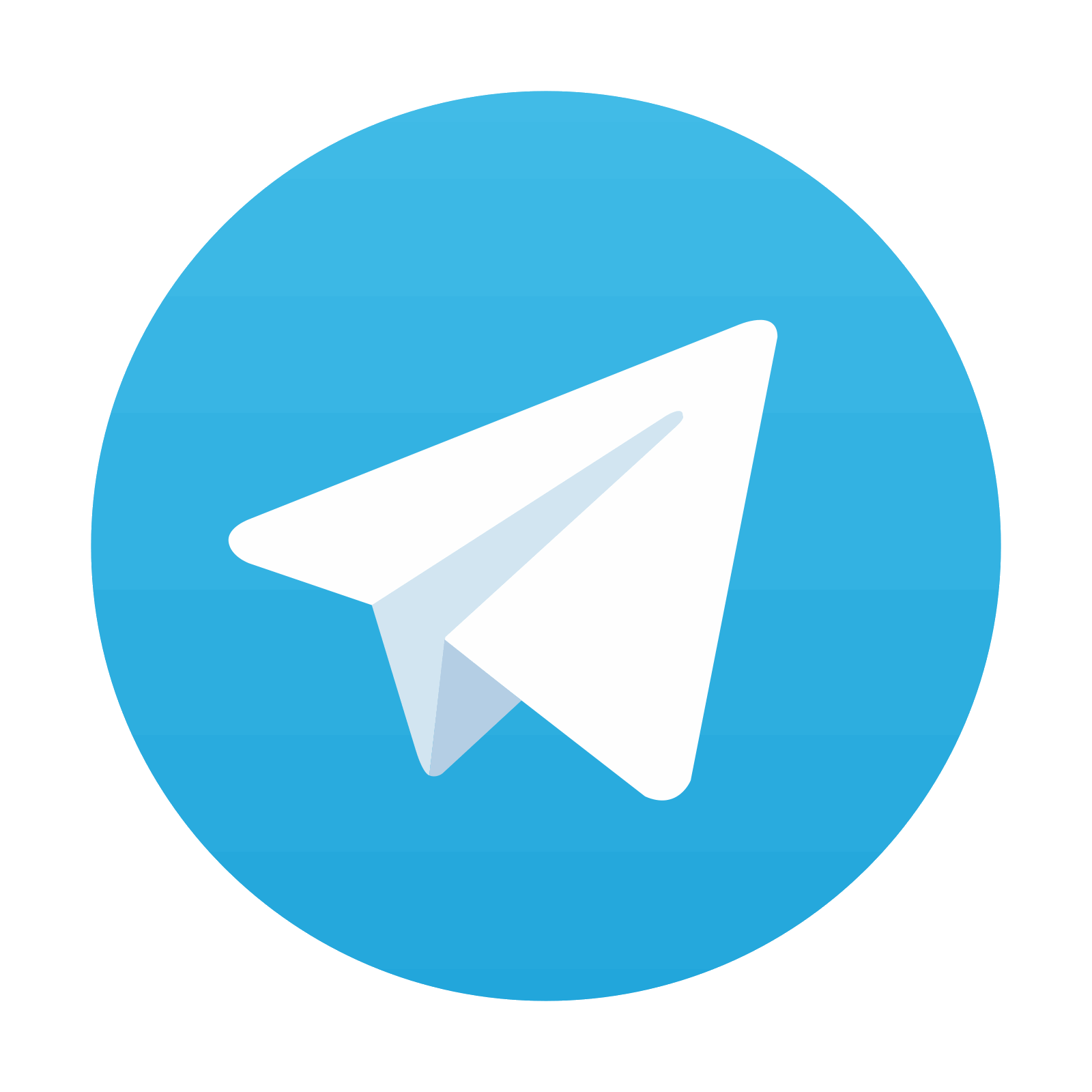
Stay updated, free articles. Join our Telegram channel
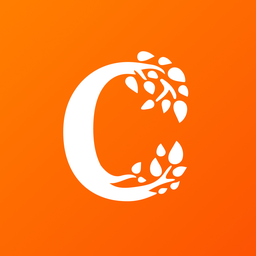
Full access? Get Clinical Tree
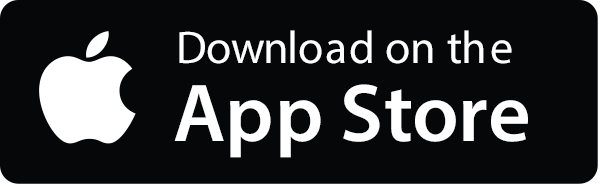
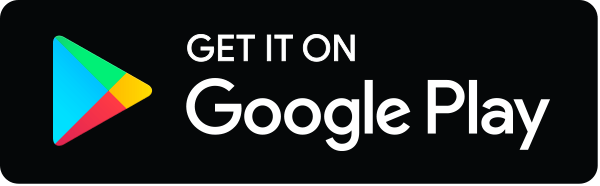