Chapter 12
Neuromuscular Blocking Agents, Reversal Agents, and Their Monitoring
History
In the nineteenth century, Claude Bernard, a famous French physiologist and philosopher, carried out experiments with curare, then in use by the Amazonian Indians of South America.1 He noted that animals the Indians hunted for food were paralyzed by arrows poisoned with curare and subsequently died of asphyxiation.2 Bernard’s experiments with the poison the Indians tipped their arrows with formed the basis for our ideas of the neuromuscular junction, neuromuscular transmission, and neuromuscular pharmacology.3 Indeed, curare had been used since 1857 as an anticonvulsant treatment in tetany and other types of spastic disorders.4
Laewen also described the use of curare in anesthetized humans in a German report in 1912.3 For readers who are interested in historical aspects of this topic, a fascinating and more complete report of the earliest work of these and other researchers, beginning as early as the year 1548, is available in an outstanding review article by Bisset.5
In 1936 Dale and colleagues found that acetylcholine (ACh) was the chemical neurotransmitter that activated the postjunctional muscle membrane receptors after excitation of the nerve terminal.5 This finding contradicted the once widely held theory that direct electrical transmission from the nerve to the muscle occurs.6 This discovery provided the impetus for further research concerning pharmacologic agents that could either enhance the action of ACh or prevent it, thereby causing a temporary and reversible state of therapeutic paralysis.
Griffith and Johnson7 of Montreal, Canada, are universally acknowledged as the persons responsible for the introduction of neuromuscular blockers into anesthetic practice. Their groundbreaking report laid the foundations for other studies that followed. Within a year of their study, Cullen reported on the use of curare in 131 general anesthetic procedures. His only report of an adverse reaction dealt with a 44-year-old woman who experienced “complete paralysis and severe salivation,” accompanied by muscular twitching.4
Despite initial successes with the neuromuscular blockers, an early study nearly doomed their use before they became widely accepted. Henry Beecher and Donald Todd, two physicians in the anesthesia department of Harvard Medical School, reviewed 599,548 anesthetic procedures administered at 10 institutions between 1948 and 1952. As part of this review, they examined the death rate in patients receiving curares (the term by which they described any neuromuscular blocking agent, including tubocurarine chloride, decamethonium bromide, succinylcholine chloride, gallamine triethiodide, and dimethyl-tubocurarine [d-tubocurarine] iodide). Beecher and Todd8 found that the overall death rate for persons treated with neuromuscular blockers was 1:370, compared with a death rate of 1:2100 in patients who did not receive these agents.
[I]n our judgment the situation is one where neither experience of individual nor experience of institution appears to protect. This adds up to evidence that neither mistakes nor preventable error of any kind are involved in the main, but rather the inherent toxicity of the “curares” themselves.8
Having presented the foregoing evidence and comment, one can ask what, then, is to be done about these agents? Are they to be banned as a practical solution of the problem? We believe not. These data strongly suggest that great caution in the use of muscle relaxants should be exercised, that the agents available at present be considered as on trial, and that they be employed only when there are clear advantages to be gained by their use, that they not be employed for trivial purposes or as a corrective for generally inadequate anesthesia.8
Beecher and Todd’s admonition still echoes through the halls of anesthetic practice today. Although the safety and efficacy of neuromuscular blocking agents have markedly increased, the sage advice is still germane for the practitioner: Neuromuscular blocking agents, like all anesthetic agents, are best used where and when they are indicated. Nevertheless, as one leg of the anesthetic objectives that includes anesthesia, analgesia, amnesia, and muscle relaxation, neuromuscular blockade has become an integral part of most modern anesthetic techniques. A broad spectrum of these agents now exists, although no single agent has all of what would be the ideal properties. Their individual pharmacokinetic and pharmacodynamic attributes enable the anesthetist to tailor the use of the agent to the physiologic needs of the patient and the requirements of the surgeon.
Monitoring of Neuromuscular Blockade
Monitoring of neuromuscular blockade should be the standard during most anesthetics when a relaxant is administered. Combining objective data from nerve monitoring with clinical signs of paralysis offers obvious advantages when dosing relaxants intraoperatively and assessing postoperative recovery. An important anesthetic discussion has been taking place on the gap between the scientific knowledge on effective monitoring and use of muscle relaxants in anesthesia and actual current clinical practice.9–12 Expert consensus strongly recommends the routine monitoring of neuromuscular block in the perioperative period as a safety issue. They feel this guides intraoperative relaxant dosing by avoiding over-paralysis and helps reduce the incidence of residual weakness in the postanesthesia care unit (PACU). A recent extensive survey of clinicians in the United States13 and Europe indicate that many practitioners are not using these monitors in everyday practice.14–16 Several explanations are given: the current quantitative monitors are cumbersome and difficult to use, qualitative monitoring is unreliable, residual paralysis is not a problem in their practice, and clinical signs can be used to assess paralysis in most patients, among others. Survey respondents felt that the incidence of residual paralysis in their practice was usually less than 1%. Objective studies estimate the incidence at about 35%.13 The discrepancy may exist because most patients recover safely in spite of residual paralysis and their problem is not noticed. Many patients may have had some difficulty or discomfort but manage to compensate until full recovery occurs and do not need specific interventions. There is no question however, that residual paralysis in the PACU represents a significant potential hazard for airway complications and aspiration. A complete discussion of neuromuscular blockade reversal is later in this chapter.
The response to a peripheral nerve monitor indirectly infers the relaxation of musculature. The neuromuscular monitor is an electrical device that delivers a series of electric shocks or impulses to the patient through electrodes applied to the skin near a nerve. There are several methods for monitoring neuromuscular blockade intensity, including acceleromyography (AMG), electromyography (EMG), phonomyography (PMG), mechanomyography (MMG), and kinemyography (KMG). Visual and tactile response to evoked electrical stimulus as assessed by the clinician, or qualitative monitoring, is the most common method. On activation of the stimulator, various predictable muscle contraction patterns are visible in the presence and absence of neuromuscular blockers. Quantitative monitoring where the stimulator is coupled with a displacement transducer as a movement measuring device and a number value is displayed is preferred but less common. Quantitative monitoring can be accomplished with MMG, AMG, KMG, or EMG.9
Depolarization and contraction of a muscle are caused by an action potential traveling along the course of a nerve. As the impulse reaches the motor endplate, acetylcholine (ACh) is released across the synaptic cleft. It subsequently travels toward the receptor sites on the muscle membrane, resulting in depolarization and subsequent contraction of the muscle.2 The stimulator elicits the same activity, which makes it useful for the monitoring of neuromuscular blockade (Figure 12-1).
Contraction of the adductor muscle of the thumb via stimulation of the ulnar nerve is the preferred site for determining the level of neuromuscular blockade. It is usually accessible and convenient. Disposable electrodes are applied over the ulnar nerve. The distal electrode is placed over the proximal flexor crease of the wrist, and the other electrode is placed over and parallel to the carpi ulnaris tendon. On stimulation of these electrodes with the monitoring device, adduction of the thumb is visible. When access to the arm and hand is not practical, other monitoring sites include the nerves of the foot and the facial nerve. Facial nerve monitoring generally involves stimulation of the temporal branch of the facial nerve that supplies the orbicularis oculi muscle around the eye or the orbicular muscle that contracts the lip.10 The facial and ulnar nerves sites are shown in Figures 12-2 and 12-3, respectively. Table 12-1 gives a comparison of the ulnar and facial nerve monitoring sites.
TABLE 12-1
A Comparison of Neuromuscular Monitoring Sites
Monitoring Site | Response | Comments |
Ulnar nerve innervation of the adductor pollicis | Thumb adduction | Usually have easy access Best site to measure recovery |
Facial nerve | Eyelid movement | Easily accessed when arm is not available Best site to measure onset |
Due to these conflicting influences, the facial nerves should be used when assessing relaxant onset. Blood, thus drug, distribution to the facial muscles mirrors distribution in the larynx and diaphragm where relaxation is required for intubation and airway manipulation. Recovery is best measured in the hand. The hand muscles are more sensitive to relaxant than the diaphragm, so if recovery is evident in the hand, the larynx and diaphragm will be recovered as well.17
Tests of Neuromuscular Function
There are five clinical tests of neuromuscular function: single twitch, train-of-four (TOF), double-burst stimulation (DBS), tetanus, and posttetanic count (PTC). The TOF, DBS, and tetanic stimulation are the most commonly used.18 The ability to evaluate muscle function with each mode of stimulation varies (Table 12-2).
A brief explanation of the concept of fade is required to understand the responses to nerve monitoring. The inability to sustain a response, known as fade, to repetitive nerve stimulation is seen in several of the clinically used monitoring tests. This is a sign of drug-induced muscle paralysis. Fade occurs because the nondepolarizing drugs block presynaptic acetylcholine receptors in addition to their classic antagonist effect at postsynaptic ACh neuromuscular junction sites. The function of presynaptic ACh receptors is to facilitate the release of ACh from the nerve terminal via a positive feedback mechanism. During high impulse rates of nerve stimulation this positive feedback mechanism prevents the decrease (fade) of transmitter release. The facilitated ACh release associated with this positive feedback mechanism is blocked by the relaxants.19 This inhibition is detected as fade during the use of the monitoring tests as described below.
Single Twitch
Unless you have a comparison twitch response before any relaxant is given, this test simply indicates whether 100% paralysis is present. If the patient’s muscle moves when stimulated, less than 100% muscle paralysis exists. If no movement is detected, 100% paralysis is present.
Train-of-Four
The second and most widely used means of stimulation is the train-of-four (TOF), which delivers four separate stimuli every 0.5 second at a frequency of 2 (Hz) for 2 seconds.20 A comparison is made of the four stimulated responses. Each of the four twitch responses are referred to as T1 through T4, respectively. Upon the onset of paralysis with a nondepolarizing relaxant, there is a progressive diminution of the twitch responses with visible fade. Fade refers to the fact that each of the successive twitch responses from T1 through T4 is smaller. When partial paralysis is present yet all four twitch responses can be elicited, with fade from T1 through T4, an assessment can be made regarding the size of T4 compared to T1. This T4:T1 ratio is referred to as the train-of-four ratio, or TOFR. Train-of-four testing can aid in approximating the degree or percent of paralysis present. It is most sensitive between 70% and 100% paralysis. The fourth twitch (T4) disappears first, which represents a block of 75% to 80%. Progressive disappearance of the third twitch (T4 and T3 absent) indicates 80% to 85% block. When three twitches are abolished (T4, T3, and T2 are absent), 90% to 95% neuromuscular blockade is present. When 100% paralysis is achieved, no responses can be elicited. Figure 12-4 correlates the responses to TOF stimulation with the approximate degree of paralysis present. Intraoperatively, the ideal degree of paralysis necessary for any procedure with sufficient anesthetic depth is 85% to 95%. That correlates with 1 to 2 twitch responses present upon TOF stimulation. Avoiding total 100% paralysis intraoperatively ensures a successful operative procedure while avoiding overdosing of the relaxant. Less total relaxant administered lessens the chances of residual paralysis upon reversal.20
A representative assessment of the TOF test during onset and recovery of a nondepolarizing relaxant is shown in Figures 12-5 and 12-6, respectively.
FIGURE 12-5 Characteristic train-of-four response during onset of a nondepolarizing muscle relaxant.
Double-Burst Stimulation
Double-burst stimulation was conceptualized as an analog to TOF with some improvements. It consists of two short bursts of a 50-Hz tetanus separated by 0.75 seconds. The use of DBS seems to improve the ability to detect residual paralysis during recovery. The suggestion is that DBS relies on the direct comparison of the muscle contraction in response to two rapidly sequential minitetanic bursts rather than the indirect comparison of the fourth twitch with the first twitch as in the TOFR. It is thought that the comparison of the fourth to the first twitches when assessing the TOFR is hindered by the second and third twitches that provide no useful information. Evaluating two rather than four twitch responses facilitates detection of fade. Tactile evaluation is suggested to improve the ability to detect fade. Responses are similar to the TOF. Fade of the second impulse is comparable to a TOFR of less than 0.6 and indicates significant paralysis.17 A comparison is shown in Figure 12-7.18,21,22
Tetanus
Tetanus consists of continuous electrical stimulation for 5 seconds at 50 or 100 Hz. The 100-Hz current is more reliable for detecting fade but is not always specific.17,23 If the muscle contraction produced is sustained for the entire 5 seconds of stimulation without fade, significant paralysis is unlikely. If fade is present, clinically significant block remains.18,24 The higher intensity of stimulation produced by tetanic frequencies as compared to TOF, DBS, or a single twitch makes it useful when other tests such as TOF or DBS are equivocal. The test is painful and should not be repeated too often to avoid muscle fatigue.
Posttetanic Count
The posttetanic count (PTC) is rarely used clinically but will be described for completeness. It is only performed when there is no response to any of the commonly used tests due to presence of 100% paralysis. Because you are already aware that the patient is completely paralyzed, the value of the PTC is to attempt to give a rough time estimate as to when recovery may occur. The PTC mode involves the use of a 50-Hz tetanic stimulation for 5 seconds, followed in 3 seconds by a series of single 1-Hz twitch stimulations.22 An understanding of the phenomenon of posttetanic potentiation (also referred to as posttetanic stimulation or facilitation) is necessary to comprehend the PTC test. When the 50-Hz tetanus is applied, there is no response because the patient is completely blocked. Application of the 50-Hz tetanus, however, transiently mobilizes excesses of acetylcholine so that after a 3-second pause, you are able to produce a short series of single-twitch responses. Because they only occur after a tetanic stimulation and not before, this single-twitch response is termed posttetanic potentiation. This extra acetylcholine will in effect “transiently reverse” the relaxant by competing for the receptor at the local monitoring site. This augmented response will only last several seconds until the excess acetylcholine dissipates. The number of twitches elicited is counted. The higher the count, the less intense the block. The usual count is between 0 (deep block) and 8 (less intense block where TOF response should return). With rocuronium, for example, neostigmine reversal of an intense block where the PTC was 1 to 2 takes more than 50 minutes.25 At a PTC of 6 to 8, reversal should occur in less than 10 minutes.
Neuromuscular monitoring terminology and tests are summarized in Box 12-1, Figure 12-2, and Table 12-3. Some key points related to successful use of tests of neuromuscular transmission are given in Box 12-2.26
TABLE 12-3
Key Points Related to Tests of Neuromuscular Transmission and Reversal
Modified from Miller RD. Neuromuscular blocking drugs. In: Miller Rd, Pardo M: Basics of Anesthesia. 6th ed. Philadelphia: Saunders; 2011:158.
Depolarizing Agents
Stephen Thesleff at the Karolinska Institute in Stockholm was one of the pioneers who introduced the drug into clinical practice to induce neuromuscular paralysis in humans. Initial description of the neuromuscular blocking properties of succinylcholine is credited to Daniel Bovet. (Bovet was awarded the Nobel Prize for Physiology and Medicine in 1957 for his discovery of synthetic compounds that act on the vascular system and skeletal muscle.)27 The first use of succinylcholine in the United States occurred in 1952. Foldes et al.28 described this agent in the following manner:
Compared to other muscle relaxants used in anesthesiology, succinylcholine possesses several advantages, the outstanding one, in our experience, being its easy controllability, which permitted almost instantaneous changes in degree of muscular relaxation. With succinylcholine, both increasing and decreasing muscular relaxation took less than a minute.28
The disadvantages of succinylcholine have been well recorded through years of clinical experience. It has no action in the ganglionic nicotinic receptors but may cause bradycardia by an action on cardiac cholinergic muscarinic receptors.29,30 Prolonged neuromuscular blockade can result from excessive doses of succinylcholine in patients with atypical, inhibited, or deficient levels of plasma cholinesterases.3,29
Succinylcholine is the only remaining depolarizing muscle relaxant licensed for use in the United States. Remembering the composition of the drug is helpful in better understanding its effects and side effects. Succinylcholine results from the joining of two ACh molecules and is represented by the chemical formula C14H30N2O4. Although succinylcholine mimics the action of ACh by depolarizing the motor endplate, its degradation is distinct. In contrast with the degradation of ACh by acetylcholinesterase (AChE), succinylcholine is hydrolyzed by plasma cholinesterase (pseudocholinesterase). The popularity of this muscle relaxant is rooted in its unique ability to provide a quick onset and short duration of effect. A bolus of 0.5 to 1.5 mg/kg is the recommended dose for adequate adult paralysis and relaxation for intubation.31 The dose of succinylcholine that provides the desired paralytic effect in 95% of the population (ED95) is approximately 0.30 mg/kg.32
Pharmacokinetics
Onset
Succinylcholine has an extremely rapid onset and remains the gold standard against which other agents are compared. In general, muscle relaxants exhibit an inverse relationship between potency and onset speed. The lower the potency, the faster the speed. A lower potency drug requires larger doses so a muscle gradient will be achieved more quickly.33 A typical intubating dose of 1 to 1.5 mg/kg results in a maximum suppression of muscle twitch and good to excellent intubating conditions within 1 to 1.5 minutes of administration.34,35 Onset of action of succinylcholine at the larynx with administration of 1 mg/kg is 34 seconds.36,37 The onset as measured at peripheral sites such as the adductor pollicis is slightly longer at 1 minute.38 The rapid onset of succinylcholine is based on its action as an initial agonist at the nicotinic receptor, rather than as a competitive antagonist. Succinylcholine works by activating the muscle-type nicotinic cholinergic receptors, followed by desensitization.30 This action results in the need for significantly less drug at the receptor site to produce neuromuscular block. In contrast, most NMBAs require 75% or more receptor occupancy for clinically useful paralysis to result. Variable onset must be considered in patients with altered physiology. Patients with atypical plasma cholinesterase may exhibit prolonged onset after succinylcholine administration.39 A summary of the dose, onset, and duration of the neuromuscular blocking drugs is given in Table 12-4.
Duration
The plasma half-life of succinylcholine is 2 to 4 minutes.40 The clinical duration of succinylcholine (i.e., the length of time during which its clinical effects can be recognized) is 5 to 10 minutes, with full recovery evident at 12 to 15 minutes. Twitch recovery of 25%, as measured by the laryngeal adductor pollicus responses, is 4.3 minutes, whereas 90% to 95% twitch recovery has been reported to occur in 8 minutes.36 Other studies have yielded similar results, with researchers citing a range of duration of 7 to 12 minutes.29,34
Elimination
Succinylcholine is degraded via hydrolysis by plasma cholinesterases. These enzymes, although found in the plasma, are produced by the liver. Initially, hydrolysis results in the transformation of succinylcholine into succinylmonocholine and choline (Figure 12-8). Succinylmonocholine is further degraded by plasma cholinesterase into succinic acid and choline. Succinylcholine metabolism is so rapid that only 10% of the injected dose ever reaches the neuromuscular junction.31 A summary of the elimination routes for the neuromuscular blocking drugs is given in Table 12-5.
TABLE 12-5
Neuromuscular Blockers: Elimination Mechanism
Agent | Elimination Mechanism | Comments |
Atracurium | Hofmann elimination; nonspecific esterases | Non–organ-dependent elimination produces consistent duration in patients with significant hepatic and renal disease, as well as the elderly |
Cisatracurium | Hofmann elimination; nonspecific esterases | Similar to atracurium but without the histamine release |
Rocuronium | Renal; hepatic | May be prolonged with hepatic and renal disease |
Vecuronium | Renal (20%-30%); hepatic (40%-80%) | May be prolonged with hepatic disease |
Pancuronium | Renal primarily; some hepatic | May be prolonged with renal disease |
Succinylcholine | Plasma cholinesterase | Prolonged in patients with cholinesterase deficiency |
Central Nervous System
Like all muscle relaxants, succinylcholine contains a quaternary ammonium in its structure, rendering it water soluble in the body. It does not therefore pass the blood-brain barrier and has no direct central nervous system (CNS) effects. Succinylcholine indirectly increases intracranial pressure (ICP), and therefore concern has always existed as to the appropriateness of its use in certain neurosurgical procedures and in patients with brain pathology and increased intracranial pressure.36 Research conducted in animals shows a small and transient rise of 10 to 15 mmHg for 5 to 8 minutes after administration.40 The rise is associated with an increased cerebral blood flow, muscle spindle afferent activity, and electroencephalogram arousal. Fasciculation of the neck muscles causing jugular vein stasis appears to be a factor.41 The ICP effects are blocked by pretreatment with a small dose of nondepolarizing relaxant.42 In clinical practice, the administration of succinylcholine is preceded by an anesthetic induction agent that lowers ICP, so that may help counteract this effect as well. Nevertheless, the routine use of succinylcholine in neurosurgery has declined. It remains widely used, with nondepolarizing relaxant and lidocaine pretreatment, for emergency procedures requiring rapid airway control via rapid sequence induction.29
Cardiovascular System
Succinylcholine usually results in slight tachycardia; however, sudden abrupt bradycardia may result from repeat dosing in adults and any dose in children. Many types of arrhythmias have been reported. The bradycardia results from autonomic ganglia and parasympathetic muscarinic receptor stimulation. Another possible mechanism for the bradycardia associated with succinylcholine administration is thought to be related to its metabolite, succinylmonocholine, which causes stimulation of cholinergic receptors in the sinoatrial node.43
An intubating dose of succinylcholine increases serum potassium levels by 0.5 to 1 mEq/L.40 Although this may not be significant in the normokalemic patient, it may be life threatening in patients with preexisting hyperkalemia.44 Gronert45 presents a case that involved an 11-year-old girl who experienced cardiac arrest after receiving succinylcholine. Her cardiac arrest and eventual death were directly attributed to a high potassium release after the succinylcholine administration (10.2 mEq/L). The exaggerated potassium release after the succinylcholine administration in this case was determined to be related to a familial myopathy evidenced by extremely high patient levels of creatine kinase. Succinylcholine administration, hyperkalemia, and myopathy are discussed later. Some clinicians believe that a second dose of succinylcholine indicated by any event should be preceded by intravenous (IV) atropine or glycopyrrolate for its anticholinergic effects; however, others do not.44,46
Hepatic System
Cholinesterase enzyme subtypes are produced in the liver. Pseudocholinesterase (PChE) degrades succinylcholine; therefore certain types of liver damage may prolong the effects of the drug.43 Ester compounds like succinylcholine are metabolized by adding water, the process referred to as hydrolysis. The basic reaction is ESTER + H2O ↔ ACID + ALCOHOL. Three esterase enzymes that can act as catalysts for these hydrolysis reactions exist in the plasma: cholinesterase, paraoxonase, and albumin esterase. Paraoxonase and albumin esterase are frequently referred to as nonspecific esterases. Additionally, red blood cells (RBCs) contain two esterase enzymes in their cytosol. One is referred to as RBC esterase, esterase D, or S-formylglutathione, and the other is AChE in small amounts.47
Cholinesterase is a generic term used for a family of related enzymes that hydrolyze choline esters at a faster rate than other esters under optimal conditions. The major function of cholinesterase is to terminate the action of ACh at cholinergic nerve endings in synapses or in effector organs.48 Two subtypes of cholinesterase exist in the human body, with several variations and a confusing set of names. One type of cholinesterase is acetylcholinesterase, AChE, also known as true, specific, genuine, and type I cholinesterase. This enzyme is found in erythrocytes, nerve endings, the lungs, the spleen, and the gray matter of the brain. It is a membrane-bound glycoprotein and exists in several molecular forms. The other subgroup pseudocholinesterase, PChE, also known as plasma, serum, benzoyl, false, butyryl, nonspecific, and type II cholinesterase, exists in plasma and has more than 11 isoenzyme variants. PChE is also present in the liver, smooth muscle, intestines, pancreas, heart, and white matter of the brain.
Measurements of PChE activity can serve as a sensitive measure of the synthetic capacity of the liver. In the absence of known inhibitors, any decrease in activity reflects impaired synthesis of the enzyme. A moderate decrease (30% to 50%) is seen in acute hepatitis and long-standing chronic hepatitis, whereas a severe decrease (50% to 70%) is seen in advanced cirrhosis and in some carcinomas with metastases to the liver. Decreased levels of PChE are also found in pregnant women and newborns and in patients with acute infections, pulmonary embolism, muscular dystrophy, myocardial infarction, and after certain surgical procedures.48 Essentially normal levels are noted in patients with mild cirrhosis or obstructive jaundice. Increased levels have been observed in cases of nephrotic syndrome, thyrotoxicosis, and hemochromatosis, in obese patients with diabetes, and in patients with anxiety and other psychiatric states. Patients generally develop neuromuscular symptoms at approximately 60% of normal activity, and serious neuromuscular effects are seen at approximately 20% of normal. Reference intervals are 2900 to 7100 units/L, although they vary with the analysis method.49
Table 12-6 lists some common anesthesia-related drugs that undergo hydrolysis, with the enzyme catalyst involved.
TABLE 12-6
Common Esterase-Dependent, Anesthesia-Related Drugs
Drug | Enzyme |
Succinylcholine | Pseudocholinesterase |
Ester local anesthetics: Cocaine Procaine Chloroprocaine Tetracaine | Pseudocholinesterase |
Neostigmine | Pseudocholinesterase |
Edrophonium | Pseudocholinesterase |
Atracurium | Nonspecific esterases (plasma) |
Cisatracurium | Nonspecific esterases (plasma) |
Remifentanil | Nonspecific esterases (plasma) |
Esmolol | Nonspecific (RBC esterases) plasma |
Clevidipine | Nonspecific estaerases (plasma) |
MOC—Etomidate | Nonspecific esterases (plasma) |
Genetic Variants of Pseudocholinesterase and the Dibucaine Inhibition Test
Some patients exhibit genetic variations in PChE that result in a prolonged response and apnea when the patient is exposed to succinylcholine. Although such individuals may lead a normal life in every other respect, their atypical variants of cholinesterase are unable to hydrolyze certain ester-containing drugs in the usual fashion. The most frequent variations in the PChE gene are the atypical (A) and Kalow (K) variants.50 Over 60 mutations in the coding sequence have been reported; however, most are extremely rare.49,51,52 The fluoride resistant (F), silent (S), and K variants are not tested for clinically because of assay difficulty and lack of clinical relevance. Phenotype-genotype concordance studies have recently been reported that allow detailed genetic mapping and clinical data to be evaluated.49
In the usual clinical scenario, a patient completes surgery and is unable to breathe. If succinylcholine was used to facilitate intubation, differential diagnosis leads the anesthesia provider to conclude that a potential atypical pseudocholinesterase may be present.53–55 The patient is taken to the PACU, placed on a ventilator, sedated, and monitored until the succinylcholine wears off. The patient recovers and is subsequently discharged, but prior to discharge, a blood sample is taken to perform a dibucaine inhibition test to help determine (1) whether an atypical enzyme was present and (2) the cause of the prolonged apnea. The dibucaine number and enzyme activity are both determined. By treating the patient’s serum with dibucaine and measuring the residual PChE activity compared with the PChE of an untreated sample, the metabolic sensitivity to succinylcholine can be measured. The patient is contacted post-discharge and counseled as appropriate, according to the findings. The administration of whole blood, fresh frozen plasma, or purified human cholinesterase has been suggested as a treatment for the prolonged apnea. Although they may be successful, there are additional transfusion risks and cost issues.43 It is safer to let the effects of succinylcholine dissipate on their own with sedation and ventilation as noted earlier.
Dibucaine is an amide local anesthetic that inhibits typical or usual PChE but not atypical PChE. For example, the normal dibucaine number of 80 means that 80% of the PChE activity was inhibited by dibucaine. If a dibucaine number of 20 is obtained, the patient has atypical enzyme because dibucaine did not inhibit the patient’s enzyme activity. If a patient experiences prolonged apnea after succinylcholine administration, it is imperative to differentiate between an atypical genetic variant of PChE or simply low levels of normal PChE enzyme. Possible interpretations of a dibucaine test are given in Box 12-3.
Patients with acute or chronic liver disease, organophosphate poisoning, or chronic renal disease, patients in the late stages of pregnancy, and those undergoing estrogen therapy may have markedly decreased PChE activities but normal enzyme. PChE phenotype interpretation is based on the total PChE activity and the percent of inhibition caused by dibucaine (Table 12-7).
Gene sequencing combined with biochemical testing can provide patients and their families with a comprehensive assessment of the likelihood of this type of event.49,50,52
Renal System
Succinylcholine may be used in surgical patients with renal disease when preoperative potassium levels are normal. The use of succinylcholine in patients with elevated preoperative potassium levels is contraindicated.56 Patients with renal failure and end-stage renal disease are frequently dialyzed prior to surgery, so as long as the serum potassium is within normal limits, succinylcholine may be used safely.57 Patients with renal disease may have lower cholinesterase levels.58
Effects in Special Populations
Elderly Patients
The onset of succinylcholine may be slightly prolonged in the elderly due to a slower circulation time, but the clinical relevance is minimal.59 Reduced plasma cholinesterase levels in elderly men may allow for a reduced dose of succinylcholine. A unique possible drug interaction has been noted in elderly patients taking tacrine (Cognex), donepezil (Aricept), rivastigmine (Exelon), or galantamine (Razadyne). These are anticholinesterase drugs used to treat Alzheimer’s disease and select forms of dementia. They increase acetylcholine levels in the CNS and are referred to as cognitive enhancers. Theoretically, the inhibition of cholinesterase produced by these agents may prolong the action of succinylcholine. The amount of systemic cholinesterase inhibition, however, does not produce clinically significant prolongation of succinylcholine60 (Box 12-4).
Obese Patients
No contraindication to the use of succinylcholine exists in obese patients. The utility of rapid-sequence induction in anesthetic management of the obese patient makes its use common.61 The recommended dose of succinylcholine is 1.0 mg/kg, based on total body weight, to produce excellent intubating conditions.62 In a 2003 study by Brodsky and Foster,63 succinylcholine was given to 14 morbidly obese patients (body mass indices ranging from 35.8 to 58) who underwent laparoscopic gastric bypass surgery. The authors administered doses ranging from 120 to 140 mg and successfully intubated all of the patients. Only 2 of the 14 patients complained of postoperative myalgia. Dosing should be based on total body weight. Because succinylcholine is water soluble, it would seem that doses based on lean body weight would suffice. Morbidly obese patients have increased fluid compartments and pseudocholinesterase levels, however, and require higher doses to ensure adequate paralysis.64,65
Pediatrics
In children, succinylcholine is used only in emergency situations to secure an airway. Routine use in elective procedures was abandoned in the early 1990s, owing to several widely reported cases of severe hyperkalemia and rhabdomyolysis in what appeared to be healthy children. The cases involved routine procedures in children with undiagnosed Duchenne muscular dystrophy (DMD).66–70 This is an X chromosome–linked disorder with onset of symptoms usually around 5 years of age. Patients exhibit a typical progression of weakness and atrophy that starts in the legs and pelvis, spreads to the shoulders and neck, and finally involves the upper extremities and respiratory muscles. Life expectancy is rarely more than 30 years; death is often a consequence of cardiac and respiratory diseases. Children with DMD frequently require orthopedic surgery for repair of scoliosis or contractures.71,72
RISK OF CARDIAC ARREST FROM HYPERKALEMIC RHABDOMYOLYSIS.
Since there may be no signs or symptoms to alert the practitioner to which patients are at risk, it is recommended that the use of succinylcholine in children should be reserved for emergency intubation or instances where immediate securing of the airway is necessary (e.g., laryngospasm, difficult airway, full stomach) or for intramuscular use when a suitable vein is inaccessible.73
Because neither succinylcholine nor halothane is routinely used in children, the incidence of masseter spasm and malignant hyperthermia has decreased. Older studies have been reported in which jaw-opening ability and the presence or absence of masseter spasm, often considered a precursor of malignant hyperpyrexia, were studied. Research was conducted on 63 children anesthetized with halothane, then relaxed with succinylcholine, pancuronium, or vecuronium. Although vecuronium and pancuronium did not cause problems with jaw opening, succinylcholine was associated with this problem, and some of the succinylcholine patients were difficult to intubate.73 Masseter spasm was noted to be more frequent in children administered succinylcholine concomitantly with halothane, compared with children who received succinylcholine and thiopental.33,74
A random sample of 6500 anesthetic records (53% of 12,169 anesthetic procedures performed) was reviewed. Fifteen cases of masseter spasm were identified. In each case, the patient underwent halothane induction and was then given succinylcholine intravenously. Seven of the 15 cases of masseter spasm developed in children between ages 8 and 10 years.75 Researchers noted an increased incidence of masseter spasm in children with strabismus who were anesthetized with halothane and received IV succinylcholine. Of 1468 halothane anesthetic procedures, 15 cases of masseter spasm were discovered, and of these 15 cases, 6 occurred in the 211 patients with strabismus.76
In current practice, masseter spasm, although rare, is seen in adults during anesthesia induction and often in emergency rooms or critical care units during emergency airway management.77,78 Common side effects of succinylcholine and contraindications for its use are noted in Table 12-8 and Box 12-5, respectively.
TABLE 12-8
Side Effects of Succinylcholine
Side Effect | Probable Cause |
Hyperkalemia | Normally, serum K+ is increased by up to 0.5 mEq/L secondary to potassium leaking from the depolarized muscle; in up-regulated patients, levels may rise much higher |
Dysrhythmias | Tachycardia (usually mild) is the most common effect; bradycardia secondary to hyperkalemia, especially with repeat doses, can occur (Wide electrocardiographic complexes leading to cardiac arrest have been seen in children with Duchenne muscular dystrophy and other muscle disorders) |
Myalgia | Secondary to fasciculation, even though some patients complain of muscle pain without having shown visible evidence of fasciculation |
Myoglobinemia | Rare complication after extensive fasciculation or in malignant hyperthermia |
Elevated intragastric pressure | Secondary to transient contraction of abdominal muscles during fasciculation; however, elevations of intragastric pressure seen after succinylcholine are not clinically relevant; less significant than occur with CO2 insufflation during laparoscopic procedures |
Elevated intracranial pressure (ICP) | Postulated to be secondary to fasciculation, increased central venous pressure; associated with increased cerebral blood flow secondary to muscle-spindle afferent activity and actions on peripheral neuromuscular junctions (ICP effects can be blocked by pretreatment with a small dose of nondepolarizing relaxant and the usual initial administration of an induction agent; may safely be used in neurosurgical procedures) |
Elevated intraocular pressure (IOP) | Increases IOP within 1 minute and peaks at an increase of 9 mmHg within 6 min after administration; increase is a vascular event, with choroidal vascular dilation or a decrease in drainage secondary to elevated central venous pressure, temporarily inhibiting the flow of aqueous humor through the canal of Schlemm; generally considered safe in ocular emergencies |
Malignant hyperthermia | Associated with a genetic predisposition; mechanism by which succinylcholine triggers the syndrome is not understood |
Masseter spasm | Seen in adults in anesthetic and emergency use; sometimes followed by malignant hyperthermia |
CO2, Carbon dioxide; K+, potassium.
Modified from Kirby RR, et al, eds. Clinical Anesthesia Practice. 2nd ed. Philadelphia: Saunders; 2002; Atlee JL. Complications in Anesthesia. 2nd ed. Philadelphia: Saunders; 2007.
Other Factors
Intraocular Pressure
Intraocular pressure (IOP) is known to increase by 5 to 15 mmHg for as much as 10 minutes after succinylcholine administration.40,79 The average is about 10 mmHg for approximately 6 minutes. The exact mechanism of this increase is unknown. Some feel that tonic contractions of the extraocular muscles via fasciculation may explain this IOP increase. It is now thought, however, that succinylcholine-induced IOP increase is a vascular event, with choroidal vascular dilation or a decrease in drainage secondary to elevated central venous pressure temporarily inhibiting the flow of aqueous humor through the canal of Schlemm.79
This rise in IOP with succinylcholine administration is significantly less than the IOP increase associated with coughing or bucking. Patients who receive succinylcholine and are intubated 1 minute after its administration had IOPs that were not significantly higher than baseline. There are no documented reports of the extrusion of globe contents following the use of succinylcholine in open-eye emergency procedures. A recent review has essentially refuted the issue of eye damage after succinylcholine administration in open-globe injuries. It appears as a theoretical but not a clinical concern. Securing the airway remains the primary issue.33,40,79 A thorough discussion of the use of succinylcholine and eye injuries is found in Chapter 39.
Hyperkalemia
Succinylcholine administration results in a transient hyperkalemia. A 0.5- to 1-mEq/L increase in serum potassium levels within 3 minutes after administration is usual. The effects were reported as lasting fewer than 10 to 15 minutes.56
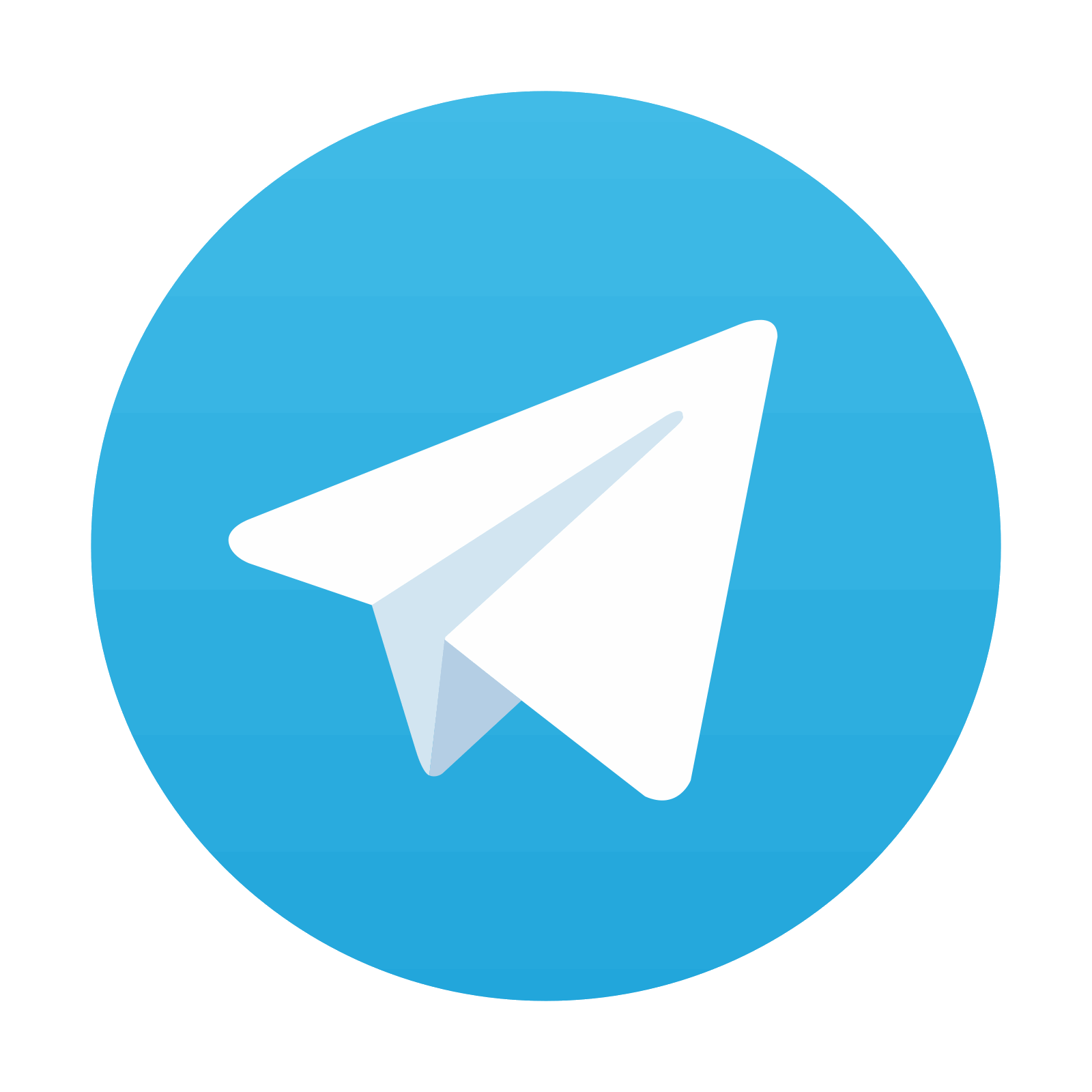
Stay updated, free articles. Join our Telegram channel
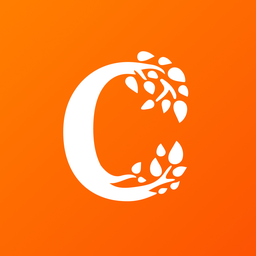
Full access? Get Clinical Tree
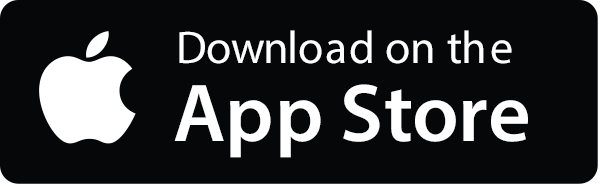
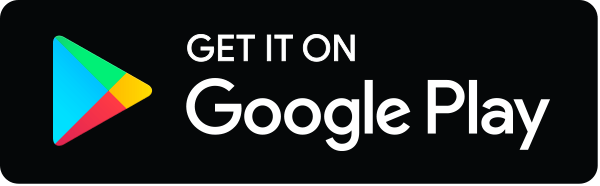
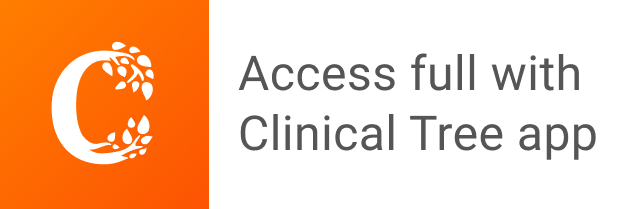