Key Points
- ▪
There are four key principles of intraoperative neurologic monitoring.
- ▪
The pathway at risk during the surgical procedure must be amenable to monitoring.
- ▪
The monitor must provide reliable and reproducible data.
- ▪
If evidence of injury to the pathway is detected, there must be some intervention possible.
- ▪
If changes in the neurologic monitor are detected, and no intervention is possible, although the monitor may be of prognostic value, it does not have the potential to provide direct benefit to the patient from early detection of impending neurologic injury.
- ▪
- ▪
There are few randomized prospective studies evaluating the efficacy of neurologic monitoring modalities.
- ▪
Anesthesiologists can improve the efficacy of monitoring by maintaining good physiologic homeostasis and stable levels of anesthesia during parts of the surgical procedure that place the nervous system at greatest risk.
- ▪
Based on clinical experience and nonrandomized studies, four practice patterns for use of neurologic monitoring have emerged:
- ▪
There are procedures for which monitoring is recommended and used by most centers.
- ▪
There are procedures for which monitoring is used frequently in some centers, but not in others.
- ▪
There are procedures for which there is no clear clinical experience or evidence indicating that monitoring is useful at all (experimental use).
- ▪
There are procedures in which monitoring is used selectively for patients believed to be at higher-than-usual risk for intraoperative neurologic injury.
- ▪
- ▪
Good communication between surgeon, anesthesiologist, and neurophysiologist is essential to optimizing the utility of monitoring.
Neurologic monitoring during anesthesia care for a patient spans a wide spectrum of techniques, diverse procedures, and various intraoperative or even postoperative settings. Techniques for monitoring fall into two broad categories: techniques to assess metabolic integrity of the nervous system, which typically entail either global or regional determinations of blood flow or oxygenation; or techniques to assess functional integrity, which likewise may be global or focused on specific anatomic pathways or structures of the nervous system. Neurologic monitoring implies that the data for the assessment of the integrity of the nervous system are acquired on a continuous or frequent intermittent basis rather than just at the beginning and end of a procedure.
The procedures and settings in which neurologic monitoring is typically applied all share the characteristic that changes in the monitored parameters can be corrected or minimized by either modifying the surgical approach or manipulating parameters under the control of the anesthesiologist. Monitored procedures range from procedures where monitoring dictates the surgical approach, such as localization of the motor strip during tumor surgery or the neurologic examination during an “awake” craniotomy, to procedures that by their nature put parts of the nervous system at increased risk.
In many procedures that require neurologic monitoring, the anatomical target is also susceptible to drugs administered in the course of an anesthetic. The anesthesiologist and the surgeon need to be aware not only of limitations inherent in individual monitoring techniques, but also of nonsurgical factors that influence the monitoring results. The monitoring approach ideally should anticipate nonsurgical factors by providing a degree of redundancy that helps distinguish a localized surgical trespass from a systemic event.
The application of neurologic monitoring to a surgical procedure may be organized in different ways. It may simply consist in setting up a piece of monitoring equipment, such as a facial nerve monitor that provides acoustic feedback to the surgeon. At the other end of the spectrum, it may require the dedicated services of a technologist and a neurophysiologist in addition to the surgical and anesthesia teams as would be the case during the mapping phase of the resection of a brain tumor. Between those extremes are a variety of delivery models for intraoperative neurophysiologic monitoring that may entail components of telemedicine. Regardless of the organizational structure, the utility of intraoperative neuromonitoring will depend on a shared understanding of surgical objectives, anesthetic constraints, and limitations of monitoring. This shared understanding will then need to be supported by open lines of communications by all involved, particularly during critical phases of an operation.
For some procedures, neurologic monitoring is a marker of the quality of care and is routinely employed because outcome data support its use. Examples include correction of scoliosis and resection of vestibular schwannomas. More frequently, the approach to monitoring is based on local conventions and surgical expectations. In this latter case, monitoring utility depends even more on a good understanding of the technique’s capabilities and limitations by anesthesiologists, surgeons, and the intraoperative monitoring team; on good communication; and on their mutual collaboration to allow corrective action in the face of changing signals or to prevent false alarms that disrupt surgery.
This chapter first discusses individual monitoring modalities in isolation so that the clinician can appreciate the inherent strengths and weaknesses of each. Subsequent sections apply this information by describing suitable approaches to various clinical settings that combine and integrate individual techniques to optimize neurologic outcome for patients. The chapter ends with a brief discussion of how neurologic monitoring is believed to be useful today, and where more work is needed to determine whether monitoring has a role in surgical patients in the future.
Monitoring Modalities
Monitors of Adequacy of Nervous System Blood Flow
Adequacy of cerebral blood flow (CBF) can be monitored by two principal methods. The first method assesses blood flow itself with the implicit assumption that “normal” flow provides adequately for the metabolic needs of the brain. The second approach assesses oxygen delivery either locally or globally with the implicit assumption that normal oxygen delivery at the site of measurement reflects adequate blood supply throughout the central nervous system (CNS). To illustrate the limitations imposed by such implicit assumptions, let us examine global or hemispheric CBF in the context of a patient’s disease process.
In normal brain, values of hemispheric CBF of approximately 50 mL/100 g/min reflect adequate oxygen delivery for maintaining structural integrity and function. Values of less than 20 to 25 mL/100 g/min are associated first with failure of function and on further decrease with structural damage. In neurosurgical patients, structural integrity and function may be altered by disease processes and anesthetics, which have an impact on the interpretation of measured CBF. A CBF of 40 mL/100 g/min in a patient in barbiturate coma after resection of an arteriovenous malformation may represent hyperemia (because metabolic demand is very low), whereas the same CBF in a patient with a mass lesion may reflect a modest decrease in cerebral perfusion pressure secondary to increasing intracranial pressure. Thus the clinical response to an abnormal value requires context and clinical judgement.
Global Blood Flow Monitoring Techniques (Noninvasive)
Intravascular Tracer Compounds
Direct measurement of CBF is possible by determining kinetics of wash-in and/or wash-out of an inert tracer compound, a method originally described by Kety and Schmidt. A widely used modern variation of the same concept is the imaging of the first passage of intravascular contrast agents during computer tomographic or magnetic resonance imaging for determining loco-regional blood flow ( Fig. 39.1 ). These techniques share the limitation of providing a snapshot of CBF in time instead of continuous assessment of flow over time.

Transcranial Doppler Ultrasound
Transcranial Doppler (TCD) ultrasound is a technique that infers CBF from measurements of the blood flow velocity in the large conducting arteries of the brain. The TCD probe transmits pulses of sound waves through the thin temporal bone in a variation of the pulsed wave Doppler technique with which anesthesiologists may be familiar from echocardiography. When these sound waves are reflected off the red blood cells back toward the TCD probe, the velocity of the reflected sound waves is changed because the blood cells themselves are in motion toward or away from the probe. This phenomenon is known as the “Doppler shift,” and is directly related to flow velocity and flow direction of the blood cells. Blood flow is faster during systole and in the center of a vessel whereas it is slower in diastole and near the vessel wall. TCD records a spectrum of flow velocities whose outline resembles an arterial waveform tracing. These concepts are illustrated in Fig. 39.2 .

Intraoperatively, TCD measurements are most commonly and easily made by continuous monitoring of the middle cerebral artery for the purpose of detecting either significant changes in flow velocity or the presence of particulate emboli. As a diagnostic study, segments of all proximal intracranial arteries and the internal carotid artery of the neck can be insonated. An important limitation of TCD results from the fact that most of the examination is done through the temporal bone, which may be thick enough to preclude an adequate examination in 10% to 20% of patients.
Two assumptions that are intuitive and plausible, but ultimately unproven, must be made for TCD-measured blood flow velocity to have a direct relationship to CBF. First, blood flow velocity is directly related to blood flow only if the diameter of the artery where the flow velocity is measured and the measurement angle of the Doppler probe remain constant. In practical terms, the difficulty with this assumption lies in finding a means to affix the TCD probe in a way that prevents dislodgment or movement during monitoring. The second assumption requires that CBF in the basal arteries of the brain is directly related to cortical CBF. Because TCD monitoring is typically done using the middle cerebral artery, this assumption may be invalid if collateral blood flow by leptomeningeal collaterals from anterior and posterior cerebral artery territories is adequate. Although these two assumptions constrain the utility of TCD as a stand-alone monitor of CBF, the changes in flow velocity seen in typical applications (discussed subsequently) are large enough to provide useful clinical information.
More importantly, TCD is the only continuous neurologic monitoring technique that provides early warning for hyperperfusion and for the number of emboli delivered to the brain during various phases of an operation. Because of their high echogenicity, emboli show up in the TCD spectrum as high-intensity transient signals (see Fig. 39.2 ) and are easily identified as brief beeps or chirps within the background of the Doppler sounds.
Jugular Bulb Venous Oxygen Saturation
The degree of oxygen extraction by an organ can be monitored by following the oxygen saturation of the mixed venous blood that drains that organ. In the case of the brain, jugular bulb venous oxygen saturation (Sjvo 2 ) is believed to measure the degree of oxygen extraction by the brain and to represent the balance between cerebral oxygen supply and demand. To monitor Sjvo 2 , a fiberoptic catheter is placed in a retrograde fashion into the jugular bulb through the internal jugular vein under fluoroscopic guidance. Correct tip placement is crucial to minimize admixture of extracranial venous blood. To decrease the risk of complications, usually only one side is monitored.
Several theoretical limitations of the technique must be borne in mind to interpret Sjvo 2 values and trends properly. Although nearly all blood from the brain drains via the jugular veins, intracranial mixing of venous blood is incomplete and may result in differences between right-sided and left-sided measurements. The dominant jugular vein (i.e., the right for most patients) drains predominantly cortical venous blood, whereas the contralateral jugular vein drains more of the subcortical regions. Despite such regional differences, Sjvo 2 must be considered a monitor of global cerebral oxygenation because inadequate perfusion to a focal brain region may not decrease Sjvo 2 values below the normal range of 55% to 75%. Because Sjvo 2 represents the balance between supply and demand, interpretation of the absolute value of Sjvo 2 must take the clinical circumstances into account.
Cerebral Oximetry
Cerebral oximetry is a noninvasive technique that, similar to Sjvo 2 , uses reflectance oximetry to measure the oxygen saturation of the tissues underneath the sensor. Typically, two sensors are applied to both sides of the forehead. The light passes not only through parts of the frontal brain, but also through the overlying skull and scalp. Contamination of the oximetry signal by extracranial blood sources is a serious concern, although the use of two sensing diodes with different distances from the light source within one sensor patch and adjustments of the algorithm of the oximeter may minimize this problem.
Because 66% to 80% of the cerebral blood volume is venous blood, cerebral oximetry determines predominantly “local venous oxygen saturation.” The simplicity of its use, and the familiarity with the principles of treating decreases in systemic mixed venous oxygen saturation, have made cerebral oximetry a popular trend monitor in operations that potentially cause decreases in blood flow to the vessels of the head. There are some significant limitations, however, of the use of cerebral oximetry during such procedures. First, adequacy of global cerebral perfusion is inferred from measurements over the frontopolar brain. Second, normative data on normal values or expected changes for cerebral oximetry are largely absent, but preoperative application of the sensors allows the start of a trend in conjunction with a neurologic baseline examination.
An example of how these limitations play out is provided by a study of the use of cerebral oximetry during 100 carotid endarterectomies in awake patients. Cerebral oximetry was able to identify 97.4% of patients with adequate CBF as indicated by the absence of clinical symptoms. The monitor frequently indicated inadequate CBF, defined as a 20% decrease in cerebral oxygen saturation from the pre-clamp baseline, although the patient had no clinical symptoms of inadequate CBF. The false-positive rate of 66.7% may simply illustrate the fact that oxygen extraction increases before function fails. The real problem is that the lower limit for acceptable regional oxygen saturation is unknown in a large population of patients. Acceptable values may be different from patient to patient, and addition of anesthetic drugs that influence cerebral metabolism may confuse the picture further.
Tissue-Level Blood Flow Monitoring Techniques (Invasive)
Tissue-level monitoring for the brain is by definition invasive. All monitors in current clinical or research use are implanted through a burr hole, extend either into the white matter or ventricular system, and typically use a bolt for stabilization. They all share a 1% to 2% risk of bleeding, infection, or ischemia owing to the implantation procedure. A second shared feature is their limited spatial resolution (i.e., each monitoring probe monitors only a limited area of brain surrounding the probe). When these monitors were first developed, there was considerable debate regarding the optimal placement of the device given such limited spatial resolution. Based on today’s appreciation for the impact of secondary neurologic insults on the ultimate outcome, there is growing agreement that tissue-level monitoring is best performed in morphologically and functionally normal tissue that is part of the penumbra or vulnerable zone of interest.
Of the tissue-level monitors, tissue partial pressure of oxygen (Po 2 ) monitoring has undergone sufficient refinement to be in wider clinical use. Thermal diffusion blood flow measurement and laser Doppler flow measurement are experimental and not in widespread clinical use.
Tissue Partial Pressure of Oxygen Monitoring
Localized monitoring of tissue Po 2 is based on an oxygen-sensitive electrode originally described by Clark. The diffusion of oxygen molecules through an oxygen-permeable membrane into an electrolyte solution causes an electric current that is proportional to Po 2 . Currently available catheter-based electrodes placed into the subcortical white matter provide stable recording conditions over long periods.
Most of the data on brain tissue oxygen levels (P Br o 2 ) come from studies in patients with head trauma. Comparison with stable xenon CT for assessment of CBF and studies during temporal clipping in aneurysm surgery show good correlation between P Br o 2 and CBF. Similarly, the time course of changes in P Br o 2 after traumatic brain injury resembles that of CBF. Critics of the technique argue that P Br o 2 values are highly influenced by the partial pressure of arterial oxygen (Pao 2 ) and are merely an elaborate indicator of the quality of patient ventilation. This view is supported by the observation that increasing the fraction of inspired oxygen (Fio 2 ) increases P Br o 2 , but likely represents an oversimplification. Concurrent microdialysis studies have shown that increasing Fio 2 not only increases P Br o 2 , but also decreases tissue lactate levels, suggesting a true improvement in the metabolic milieu of the brain tissue itself. While decreases in P Br o 2 are associated with worse outcomes in traumatic brain injury patients, the role of P Br o 2 -directed therapy is still investigational.
Monitors of Nervous System Function
The most commonly used monitors of function are the electroencephalogram (EEG), sensory-evoked responses (SERs), motor evoked responses, and the electromyogram (EMG). The EEG is a surface recording of the summation of excitatory and inhibitory postsynaptic potentials spontaneously generated by the pyramidal cells in the cerebral cortex. The signals are very small, and each recording electrode records information both directly beneath the electrode and information volume conducted from deeper tissue. Monitoring the EEG is usually directed toward one or more of four perioperative uses. First, the EEG is used to help identify inadequate blood flow to the cerebral cortex caused by either a surgically induced or anesthetic-induced reduction in blood flow or retraction on cerebral tissue. Second, the EEG may be used to guide an anesthetic-induced reduction of cerebral metabolism either in anticipation of a loss of CBF or in the treatment of high intracranial pressure, when a reduction in CBF and blood volume is desired. Third, the EEG may be used to predict neurologic outcome after a brain insult. Finally, the EEG may be used to gauge the depth of the hypnotic state of the patient under general anesthesia (see Chapter 40 ).
More than 50 years of experience monitoring the EEG has led to many known correlations of EEG patterns with clinical states of the normal and diseased cerebral cortex. The electroencephalographer can accurately identify consciousness, unconsciousness, seizure activity, stages of sleep, and coma. In the absence of significant changes in anesthetic technique, the electroencephalographer also can accurately identify inadequate oxygen delivery to the brain (from either hypoxemia or ischemia). By using high-speed computerized EEG analysis and statistical methods, EEG patterns in the continuum from awake to deeply anesthetized are becoming, with few exceptions, much better understood. In addition, computer advances have made possible high-speed mathematic manipulation of the EEG signal to present the data in a manner more suitable to continuous trends for use during surgical or anesthetic monitoring.
Evoked potentials are electrical activity generated in response to either a sensory or a motor stimulus. Measurements of evoked responses may be made at multiple points along an involved nervous system pathway. The evoked responses are generally smaller than other electric activity generated in nearby tissue (muscle or brain) and are readily obscured by these other biologic signals. In the case of SERs, repeated sampling and sophisticated electronic summation and averaging techniques are needed to extract the desired evoked potential signal from background biologic signals. Motor-evoked responses are generally larger and commonly do not require averaging.
SERs are the most common type of evoked potentials monitored intraoperatively. During the last three decades, much research has been done on the use of intraoperative motor-evoked potentials (MEPs), and use of MEPs during both intracranial and spinal surgery is now no longer considered experimental. There are three basic types of SERs: somatosensory-evoked potentials (SSEPs), brainstem auditory-evoked potentials (BAEPs), and visual-evoked potentials (VEPs).
Electroencephalogram
Basic Unprocessed Electroencephalogram Concepts
The EEG is produced by a summation of excitatory and inhibitory postsynaptic potentials produced in cortical gray matter. Because the EEG signal is generated only by postsynaptic potentials and is much smaller than action potentials recorded over nerves or from heart muscle, extreme care must be taken when placing electrodes to ensure proper placement and excellent contact with the skin to avoid significant signal loss. Alternatively, subdermal needle electrodes may be used, particularly when sterile application of an electrode close to a surgical field is necessary. When electrodes are applied directly to the surface of the brain, impedance is minimized by close electrode contact and saturation of the area with an electrolyte solution.
EEG electrodes generally are placed according to a mapping system that relates surface head anatomy to underlying brain cortical regions. The placement pattern of recording electrodes is called a montage. Use of a standard recording montage permits anatomic localization of signals produced by the brain and allows development of normative EEG patterns and comparison of EEG recordings made at different times. The standard EEG “map” is called the 10 to 20 system for EEG electrode placement ( Fig. 39.3 ). This system is a symmetric array of scalp electrodes placed systematically based on the distance from the nasion to the inion and from the pretragal bony indentations associated with both temporomandibular joints. Based on 10% or 20% of these distances, recording electrodes are placed systematically over the frontal (F), parietal (P), temporal (T), and occipital (O) regions at increasing distances from the midline. Left-sided electrodes are given odd number subscripts, and right-sided electrodes are given even number subscripts. Increasing numbers indicate a greater distance from the midline. Midline electrodes are designated with a “z” subscript. The standard diagnostic EEG uses at least 16 channels of information, but intraoperative recordings have been reported using 1 to 32 discreet channels.

The intraoperative EEG is most commonly recorded from electrodes placed on the scalp. Recordings also may be made from electrodes placed on the surface of the brain (electrocorticography), or from microelectrodes placed transcortically to record from individual neurons (e.g., during surgery for Parkinson disease). The EEG signal is described using three basic parameters: amplitude, frequency, and time. Amplitude is the size, or voltage, of the recorded signal and ranges commonly from 5 to 500 μV (vs. 1-2 mV for the electrocardiogram signal). Because neurons are irreversibly lost during the normal aging process, EEG amplitude decreases with age. Frequency can be thought of simply as the number of times per second the signal oscillates or crosses the zero voltage line. Time is the duration of the sampling of the signal; this is continuous and real time in the standard paper or digital EEG, but is a sampling epoch in the processed EEG (see later).
Normal Electroencephalogram
Normal patterns seen on the EEG vary among normal individuals, but are consistent enough to allow for accurate recognition of normal and pathologic patterns. The usual base frequency in an awake patient is the beta range (>13 Hz). This high-frequency and usually low-amplitude signal is common from an alert attentive brain and may be recorded from all regions. With eye closure, higher amplitude signals in the alpha frequency range (8-13 Hz), seen best in the occipital region, appear ( Fig. 39.4 ). This “eyes closed” resting pattern is the baseline awake pattern used when anesthetic effects on the EEG are described. When events that lead the brain to produce higher frequencies and larger amplitudes occur, the EEG is described as “activated,” and when slower frequencies are produced (theta = 4-7 Hz, and delta = <4 Hz), the EEG is said to be “depressed.” The EEG during natural sleep may contain all of these frequencies at various times. The slower frequencies occur during deep natural sleep with “sleep spindles” ( Fig. 39.5 ), but during light sleep or rapid eye movement sleep, the EEG becomes activated, and the eye muscle EMG appears on the EEG.


In the normal EEG in awake and asleep patients, patterns recorded from corresponding electrodes on each hemisphere are symmetric in terms of frequency and amplitude, the patterns are predictable if clinical states are known, and spike (epileptic) waveforms are absent. In most cases, normal EEG patterns are associated with normal underlying brain function in awake and asleep patients.
Abnormal Electroencephalogram
General characteristics of the “abnormal” EEG include asymmetry with respect to frequency, amplitude, or both, recorded from corresponding electrodes on each hemisphere, and patterns of amplitude and frequency that are unpredictable or unexpected in the normal recording. These abnormal patterns reflect either anatomic or metabolic alterations in the underlying brain. Regional asymmetry can be seen with tumors, epilepsy, and cerebral ischemia or infarction. Epilepsy may be recognized by high-voltage spike and slow waves, whereas cerebral ischemia manifests first with EEG slowing with preservation of voltage. Further slowing and loss of voltage occurs as ischemia becomes more severe. Factors affecting the entire brain may produce symmetric abnormalities of the signal. Identifying pathologic abnormal patterns in the global EEG signal is very important, although sometimes quite difficult, in the clinical situation. Many of the normal global pattern changes produced by anesthetic drugs are similar to pathologic patterns produced by ischemia or hypoxemia. Control of anesthetic technique is very important when the EEG is being used for clinical monitoring of the nervous system.
Processed Electroencephalogram Concepts
Numerous limitations are introduced when moving from the raw EEG domain to the processed EEG domain. First, artifact is processed in many cases along with desired signal leading to a perfectly believable processed EEG display that is materially incorrect. Second, the standard 16-channel EEG montage provides more information than can be practically analyzed or displayed by most processed EEG monitors and perhaps more than is needed for routine intraoperative use. Most available processed EEG devices used by anesthesiologists use four or fewer channels of information—translating to at most two channels per hemisphere. Processed EEG devices generally monitor less cerebral territory than a standard 16-channel EEG. Third, some intraoperative changes are unilateral (e.g., regional ischemia owing to carotid clamping), and some are bilateral (e.g., EEG depression by bolus administration of an anesthetic). Display of the activity of both hemispheres is necessary to delineate unilateral from bilateral changes. An appropriate number of leads over both hemispheres is needed. Most early studies validating intraoperative EEG monitoring used continuous visual inspection of a 16- to 32-channel analog EEG by an experienced electroencephalographer—such monitoring was considered the gold standard. Adequate studies comparing the processed EEG with fewer channels with this gold standard across multiple uses and operations have not been done, although limited data using processed EEG monitoring during carotid surgery suggest that two- or four-channel instruments would detect most significant changes, provided that the electrodes are appropriately placed over watershed areas of blood supply.
Devices
EEG processing for intraoperative monitoring is typically based on power analysis of a segment of raw EEG, referred to as an epoch. Power analysis uses Fourier transformation to convert the digitized raw EEG signal into component sine waves of identifiable frequency and amplitude. The raw EEG data, which is a plot of voltage versus time, is converted to a plot of frequency and amplitude versus time. Many commercially available processed EEG machines display power (voltage or amplitude squared) as a function of frequency and time. These monitors display the data in two general forms, either compressed spectral array or density spectral array. In compressed spectral array, frequency is displayed along the x axis, and power is displayed along the y axis with height of the waveform equal to the power at that frequency. Time is displayed along the z axis. Tracings overlap each other, with the most recent information in front ( Fig. 39.6 ). Density spectral array also displays frequency along the x axis, time is displayed along the y axis, and power is reflected either by the density of the dots at each frequency or by a spectrum of colors. Each display format provides the same data, and choice depends on the preference of the user.

Many changes that occur during anesthesia and surgery are reflected as changes in amplitude, frequency, or both. These changes can be clearly seen in these displays if adequate and appropriate channels are monitored. Power analysis has been used clinically for many years as a diagnostic tool during procedures with risk for intraoperative cerebral ischemia, such as carotid endarterectomy and cardiopulmonary bypass (CPB). Power analysis has proven to be a sensitive and reliable monitor in the hands of experienced operators using an adequate number of channels. In addition, parameters obtained from power analysis have been investigated as monitors for depth of anesthesia.
Data Acquisition Period
An important consideration in the processed EEG is time. Raw EEG is continuous in real time. The processed EEG samples data over a given time period (epoch), processes the data, and then displays information in various formats. There is a relationship between epoch length and spectral resolution. If a long epoch length is chosen, the waveform can be described precisely, but the time required for data processing is long and not real time. If a short length of data is sampled, analysis may be done in near real time, but the epoch chosen for analysis may not be representative of the overall waveform (i.e., the condition of the patient). There also may be insufficient data points for meaningful Fourier transformation. This issue, as related to the use of intraoperative EEG for analysis of anesthetic depth, has been studied by Levy. A longer epoch may produce less epoch-to-epoch variability and allow more precise description of frequency and power; however, the longer epoch increases the delay before new information is processed and displayed, reducing the amount and timeliness of information available for clinical decision making. In studying EEG epochs of 2 to 32 seconds, Levy concluded that 2-second epochs are appropriate during general anesthesia. Many commercially available devices have used 2-second epoch lengths, updated at varying user-selected intervals. With better and faster computers, continuous monitoring of 2-second epochs and now even longer epochs is possible.
Evoked Potentials
Basic Concepts Common to All Modalities
EEG signals provide information about cortical function, but little to no information about subcortical neural pathways crucial to normal neurologic function. Intraoperative monitoring of SERs has gained increasing popularity over the last 35 years because it provides the ability to monitor the functional integrity of sensory pathways in an anesthetized patient undergoing surgical procedures placing these pathways at risk. Because motor pathways are often adjacent anatomically to these sensory pathways or supplied by the same blood vessels, or both, function of motor pathways may be inferred, albeit imperfectly, from the function of these sensory pathways. Today, MEPs are monitored together with SERs to provide direct information about function of motor pathways. SERs are typically 100-fold smaller in amplitude than the EEG. Recording SERs in an environment such as an operating room with its myriad electrical devices is challenging and requires substantial technical expertise.
Sensory-Evoked Responses
SERs are electric CNS responses to electric, auditory, or visual stimuli. SERs are produced by stimulating a sensory system and recording the resulting electric responses at various sites along the sensory pathway up to and including the cerebral cortex. Because of the very low amplitude of SERs (0.1-10 μV), it is often impossible to distinguish SERs from other background biologic signals, such as the EEG or EMG, which may be considered in this case undesirable “noise.” To extract the SER from the background noise, the recorded signal is digitized, and signal averaging is applied. With this technique, signal recording is time-locked to the application of the sensory stimulus. For example, during intraoperative posterior tibial nerve SER monitoring, after nerve stimulation at the ankle, only signal information occurring less than 90 ms after the stimulus is recorded ( Fig. 39.7 ). The SER occurs at a constant time after the stimulus application; other electric activity, such as spontaneous EEG, occurs at random intervals after the sensory stimulus. The averaging technique improves the SER signal-to-noise ratio by eliminating random elements and enhancing the SER. This enhancing effect increases directly with the square root of the number of responses added into the averaged response.

SER recordings are of two general types determined by the distance of the recording electrode from the neural generator of the evoked response. SERs recorded from electrodes close to the neural generators (within approximately 3-4 cm in the average adult) are termed “near-field potentials.” Near-field potentials are recorded from electrodes placed very close to the actual signal generator site, and the morphology is directly affected by electrode location. Far-field potentials are recorded from electrodes located a greater distance from the neural generator and are conducted to the recording electrode through a volume conductor (brain, cerebrospinal fluid, and membranes). Because the current spreads diffusely throughout the conducting medium, it is more difficult to locate the source of the recorded signal and the electrode position has little effect on the morphology of the recorded evoked potential (see Fig. 39.7 ). As the distance between the recording electrode and the neural generator increases, the recorded SER becomes smaller. More responses have to be averaged to record far-field potentials (several thousand) than near-field potentials (50–100).
SERs also may be described as cortical or subcortical in origin. Cortical SERs are generated by the arrival at the cortex of the volley of action potentials generated by stimulating the sensory system. Because they are recorded as a near-field potential, they are typically easy to identify by elapsed time, waveform morphology, and amplitude. Subcortical responses may arise from many different structures depending on the type of response, including peripheral nerves, spinal cord, brainstem, thalamus, cranial nerves, and others. Cortical SERs are usually recorded from scalp electrodes placed according to the standard 10 to 20 system for EEG recordings (see Fig. 39.3 ). Subcortical evoked responses also may be recorded as far-field potentials from scalp electrodes or, as appropriate, from electrodes placed over the spinal column or peripheral nerve.
Evoked potentials of all types (sensory or motor) are described in terms of latency and amplitude (see Fig. 39.7 ). Latency is defined as the time measured from the application of the stimulus to the onset or peak (depending on convention used) of the response. The amplitude is simply the voltage of the recorded response. According to convention, deflections below the baseline are labeled “positive (P),” and deflections above the baseline are labeled “negative (N).” Because amplitude and latency change with recording circumstances, normal values must be established for each neurologic monitoring laboratory, and may differ from values recorded in other laboratories.
SERs used for intraoperative monitoring include SSEPs, BAEPs, and rarely VEPs. For all these techniques, cortical recording electrodes are placed on the scalp, using the same standard 10 to 20 system as for recording the EEG, whereas recordings for subcortical and peripheral signals are placed in various standardized anatomic locations. The surgical incision and the need for sterility may necessitate nonstandard electrode placements. Such deviations must be considered when interpreting baseline and subsequent SERs. In the case of MEPs, stimulating electrodes also are placed according to the 10 to 20 system of electrode placement, but over the motor cortex instead. Recording electrodes may be placed over the spinal column, peripheral nerve, and (most commonly) innervated muscle.
One of the most important principles of recording SERs intraoperatively is that reproducible, reliable tracings must be obtained at baseline before any intervention likely to cause changes in the evoked response. If good-quality tracings with identifiable waveforms cannot be recorded and reproduced at baseline, evoked-response monitoring would be of little use in monitoring the integrity of the CNS intraoperatively. If significant variability exists, or waveforms are difficult to identify, it will be impossible intraoperatively to distinguish SER changes that are clinically significant from a preexisting baseline variability of waveforms. When good, reproducible responses cannot be recorded at baseline, monitoring should not be used for clinical decision making. It is helpful to comment on the quality of the baseline SERs during the preincision time out so that the whole team is better positioned to put changes in SERs into context.
Somatosensory-Evoked Potentials
SSEPs are recorded after electric stimulation of a peripheral mixed nerve using needles or surface gel electrodes. SSEP responses consist of short-latency and long-latency waveforms. Cortical short-latency SSEPs are most commonly recorded intraoperatively because they are less influenced by changes in anesthetic drug levels. The pathways involved in the generation of upper extremity short-latency SSEPs include large-fiber sensory nerves with their cell bodies in the dorsal root ganglia and central processes traveling rostrally in the ipsilateral posterior column of the spinal cord synapsing in the dorsal column nuclei at the cervicomedullary junction (first-order fibers), second-order fibers crossing and traveling to the contralateral thalamus through the medial lemniscus, and third-order fibers from the thalamus to the frontoparietal sensorimotor cortex. These primary cortical-evoked responses, which are recordable with most anesthetic techniques, result from the earliest electric activity generated by the cortical neurons and are thought to arise from the postcentral sulcus parietal neurons. The longer-latency secondary cortical waves are thought to arise in the association cortex. These responses have much greater variability in an awake patient, habituate rapidly on repetitive stimulation, and are only poorly reproducible during general anesthesia. Cortical SSEPs other than the primary cortical response are not monitored or interpreted intraoperatively because they are severely altered by general anesthesia.
Although most evidence indicates that upper extremity evoked potentials are conducted rostrally in the spinal cord through dorsal column pathways, some data suggest that lower extremity SSEPs are conducted at least partially by the lateral funiculus. Stimulation of the posterior tibial nerve or common peroneal nerve at or above motor threshold activates group I fibers that synapse and travel rostrally through the dorsal spinocerebellar tract. After synapsing in nucleus Z at the spinomedullary junction, the pathway crosses and projects onto the ventral posterolateral thalamic nucleus. This pathway difference is important because the dorsal lateral funiculus is supplied primarily by the anterior spinal artery, the artery that also supplies the descending motor pathway and neurons in the spinal cord. Manipulations, such as distraction of the spinal column to correct scoliosis, which may secondarily compress or distort radicular blood supply to the anterior spinal cord, should cause changes in the SSEP in the event blood supply is reduced to critical levels. This hypothesis is verified by the very low, but not zero, incidence of postoperative paraplegia on awakening without any intraoperative changes in SSEPs.
For SSEP recordings with median nerve stimulation, recording electrodes are first placed at Erb point, just above the midpoint of the clavicle. This point overlies the brachial plexus, and signals recorded here assure the clinician that the stimulus is actually being delivered properly to the patient. The next electrode is placed midline posteriorly over the neck at level of the second cervical vertebra, relatively near the dorsal column nuclei. Signals recorded here ensure proper transmission of the response from the peripheral nervous system into the spinal cord and rostral along the spinal cord to the lower medulla. The final electrodes are placed on the scalp overlying the sensory (parietal) cortex contralateral to the stimulated limb. Signals recorded here ensure the integrity of the pathway through the brainstem, thalamus, and internal capsule, and may assess adequacy of CBF in this area of the cortex.
To record SSEPs after posterior tibial nerve stimulation, electrodes are placed first over the popliteal fossa to ensure proper stimulus delivery to the nervous system. Electrodes also may be placed over the lower lumbar spine to ensure proper transmission of the signal into the spinal cord itself, but this site is not commonly used because of the proximity of sterile surgical incisions. Cervical spine and scalp recording electrodes are placed in a similar fashion as described previously, although different locations may be used as required by the surgical incision. More invasive recording methods, such as epidural electrodes, also may be used intraoperatively.
Purported generators for short-latency SSEPs are listed in Table 39.1 and shown in Fig. 39.8 . Induction of anesthesia, a patient’s neurological disease or age, and use of different recording electrode locations (montage), necessitated by the surgical incision, may significantly alter the appearance of the SSEP. In these cases, attribution of a particular generator to a given wave on the tracing may be quite difficult. During neurologic monitoring, such precision is not needed, and recorded waveforms are compared with tracings obtained at baseline and during earlier portions of the surgical procedure. After lower limb stimulation, absolute latencies are increased because of the greater distance the response to stimulation must travel along the peripheral sensory nerve and spinal cord. Interpeak latencies (see Fig. 39.7 ) also are evaluated to assess specific conduction times, such as N9 to N14 conduction time, reflecting transmission time from the brachial plexus to brainstem, or N14 to N20 conduction time, reflecting transmission time between the dorsal column nuclei and the primary sensory cortex. Latencies are also significantly affected by age at both extremes of life and many neurologic disorders.
Peak | Generators |
---|---|
N9 (EP) | Brachial plexus ∗ |
N11 | Posterior columns or spinal roots |
N13/P13 | Dorsal column nuclei ∗ |
N14, 15 | Brainstem or thalamus |
N19/P22 | Parietal sensory cortex ∗ |
∗ Indicates sites commonly recorded during surgical procedures. All other waveforms indicated are not commonly monitored.

Brainstem Auditory-Evoked Potentials
BAEPs are produced in the diagnostic laboratory by delivering repetitive clicks or tones via headphones. Headphones are not practical for surgical monitoring of neurosurgical procedures, and click stimuli are delivered using foam ear inserts attached to stimulus transducers ( Fig. 39.9 ). Stimulus intensity is usually set at 60 to 70 dB above the patient’s click-hearing threshold, although, practically speaking, many intraoperative laboratories establish monitoring after induction of anesthesia and instead begin with a stimulus intensity of 90 dB nHL (normal hearing level). The duration of the click is approximately 100 μs, and the stimulus is given usually 10 to 15 times per second. Clicks are delivered using different “polarities”—that is, the click may cause initial movement of the tympanic membrane away from the transducer (rarefaction) or toward the transducer (condensation). Use of these two different methods commonly produces very different waveforms, amplitudes, and latencies in individual patients, and the method that produces the largest reproducible response is chosen. If stimulus artifact is a serious problem, clicks of alternating polarity may be used to decrease the artifact, but the waveforms produced are an average of those produced by either stimulating technique alone and may be more difficult to monitor.

Rate and intensity of stimulus delivery affect BAEPs. Unilateral stimulation is used because responses from the other ear, which may remain normal during surgery, may obscure any abnormal responses from the monitored ear. Recording electrodes are placed on the lobe of the stimulated ear and on the top of the head (vertex). White noise may be delivered to the contralateral ear to prevent bone conduction from stimulation of the monitored ear from producing an evoked response from the contralateral ear. On average, 500 to 2000 repetitions are required because BAEPs recorded from the scalp are far-field potentials and extremely small (often <0.3 μV).
Peaks in recordings of BAEPs are labeled I through VII; the purported neural generators for these peaks and the auditory pathway are shown in Fig. 39.10 . The anatomic auditory pathway would predict that BAEP monitoring would be most useful for surgical procedures in the posterior fossa that risk hearing or structures in the upper medulla, pons, and midbrain. As with other SERs, amplitude, absolute latencies, and interpeak latencies are evaluated to assess integrity of the auditory system, localize the functional defect when it occurs, and assess peripheral and central conduction times. Because waves VI and VII are inconsistent and variable, they are not routinely monitored, and most articles reporting use of BAEP for surgical monitoring in the operating room monitor waves only up to wave V.

Visual-Evoked Potentials
VEPs are recorded after monocular stimulation with recording electrodes over the occipital, parietal, and central scalp. Flash stimulation of the retina using light-emitting diodes embedded in soft plastic goggles through closed eyelids or as needed via contact lenses with built-in light-emitting diodes is provided. VEPs are cortical SERs, which vary with the type of stimulus, part of the retina stimulated, degree of pupil dilation, and patient’s attention level. Because some of these factors change commonly and even constantly during the course of every anesthetic, VEPs would be expected to be highly variable during surgery even when no surgical trespass on the visual system occurs. VEPs are the least commonly used evoked-response monitoring technique intraoperatively. However, some investigators have recently been able to generate reproducible intraoperative VEPs using multiple red light-emitting diodes embedded in a soft silicone disk placed directly on the cornea for stimulating the retina. This technique may allow for further studies which will determine the clinical utility of this modality. However, flash stimuli generate potentials in all areas of the primary visual cortex at once making it difficult detect an injury to a small area of cortex.
Motor-Evoked Potentials
MEPs are generated most commonly by the application of a transcranial train of electric stimuli, and responses are recorded at various points along the spinal column, peripheral nerve, and innervated muscle.
Transcranial Motor-Evoked Potentials
Monitoring of the integrity of the motor tracts within the spinal cord is a technique with great potential benefit, and even during the relative short history of MEP monitoring, there are reported cases of loss of MEPs with preservation of the SSEP. This technique is used extensively in spinal surgery, in which transmission across the operative field can be assessed, and in aortic surgery, with the potential for impairment of the blood supply to the vulnerable anterior spinal cord. Relative to SER monitoring, MEP monitoring is quite invasive and, in the case of transcranial stimulation, uses much higher stimulus intensity (≥400 V). Special stimulation techniques may be used to obtain transcranial MEPs (tcMEPs) from young children or adults with some degree of neurologic compromise at baseline.
Several variants of MEP monitoring exist. The most common method involves transcranial electrical stimulation. During transcranial electric MEP monitoring, stimulating electrodes (usually small, metallic screw-type electrodes similar to those used in fetal monitoring) are placed into the scalp overlying the motor cortex, and a train of electric stimuli (usually around 400–500 V) is applied to the scalp. This stimulation definitely activates muscles of mastication, and bilateral bite-blocks must be placed to prevent serious damage to the tongue during stimulation. Alternatively, if the precentral gyrus or motor strip is exposed during surgery, stimulating electrodes may be placed directly onto the cortex. Because approximately 90% of the transcranial stimulus dissipates across scalp and skull, typical stimulus intensities of direct cortical stimulation are 40 to 50 V.
Both stimulating methods also activate surrounding cortical structures and subcortical white matter pathways (sensory and motor). In fact, tcMEPs can frequently be recorded from patients with cerebral palsy, despite the disruption of their cortical neuronal architecture. Distal antidromic propagation of the transcranially applied stimulus is blocked by synapses in all of the ascending sensory pathways. The stimulus is propagated easily orthodromically through descending motor pathways. It is important to note that only the largest diameter fibers, 2% to 3% of all fibers in the corticospinal tract, propagate the impulses associated with tcMEPs. The evoked responses may be recorded over the spinal cord, the peripheral nerve, and, most commonly, the muscle itself. To enhance the MEP, these responses may be averaged in the same manner as SERs, but averaging is almost always unnecessary. Activation of the corticospinal tract well below the motor cortex may limit the utility of tcMEP to assess the adequacy of CBF to the motor cortex, because activation may be distal to the site of ischemia and part of a different vascular bed.
Electromyography
Intraoperative monitoring of EMG responses generated by cranial and peripheral motor nerves allows early detection of surgically induced nerve damage and assessment of level of nerve function intraoperatively. In these cases, the ability of a nerve to produce a response in the innervated muscle is used to assess the health of a cranial or peripheral nerve at risk during surgery. Recordings are made from either surface (electrocardiogram or gold cup) electrodes or needle electrodes placed directly in the innervated muscle of interest. Sensitivity of EMG recordings is best if recordings are made from needles within the muscle itself. Surface electrodes and, to a lesser extent, subdermal needle electrodes may completely miss neurotonic discharges that indicate damage to a nerve. The most experience with this monitoring modality has been obtained during facial nerve monitoring.
EMG monitoring may be either active or passive. During active monitoring, a cranial or peripheral nerve is stimulated electrically, and the evoked EMG (compound muscle action potential) response from the muscle is recorded. Stimulation of the nerve proximal to the operative area or tumor can be used to assess functional integrity of the nerve. Nerve function also may be assessed by noting the intensity of nerve stimulus needed to evoke a muscle response and by the morphology of the compound muscle action potential. Nerve function may be monitored passively during surgery with continuous recording of all generated responses from innervated muscle groups. “Popcorn” EMG discharges are produced by simple, benign contact with the monitored nerve. Response trains are produced with more significant nerve irritation. Neurotonic discharges are produced by significant nerve irritation or damage or both ( Fig. 39.11 ). When these EMG responses reach a certain voltage threshold, they are usually converted into audible signals that provide immediate feedback to the surgeon and warn of impending nerve damage in real time. Real-time feedback is key because density and frequency of neurotonic discharges may correlate with degree of postoperative nerve dysfunction, as shown by data obtained from patients undergoing resection of acoustic tumors. One caveat of EMG monitoring is that sharp section of a nerve may produce no EMG discharge at all.

Intraoperative monitoring of the motor component of other cranial nerves also has been successfully performed. EMG monitoring of the trigeminal nerve can be accomplished with electrodes placed over or in the temporalis or masseter muscles. Trigeminal nerve motor monitoring has been used during nerve section for tic douloureux to ensure preservation of the motor branch of the trigeminal nerve and in combination with facial nerve monitoring during resection of large posterior fossa lesions. Using recording electrodes placed in or over the trapezius or sternocleidomastoid muscles, the spinal accessory nerve has been successfully monitored during resection of large meningiomas, glomus jugulare tumors, and neck carcinomas. EMG monitoring of the hypoglossal nerve with needle electrodes placed in the tongue has been infrequently used for large posterior fossa lesions and clivus tumors. EMG monitoring of the eye muscles can be performed using tiny hook wires for recording; however it is rarely used in practice.
Monitoring of peripheral motor nerves has been performed by placing needle electrodes in or over the muscles innervated by nerves that traverse the operative area and are at risk from the planned surgical procedure. Auditory feedback from EMG monitoring can warn the surgeon of unexpected surgical trespass of the nerve, help locate a nerve within the field (e.g., during untethering of the spinal cord), and localize the level of any conduction block or delay. Because radiculopathies have been reported to occur after spine surgery, particularly because of incorrect pedicle screw placement, EMG monitoring of peripheral nerves has been used in patients undergoing spine surgery to decrease the risk of nerve root injury during the procedure. During pedicle screw placement, the surgeon can stimulate the screw directly with a small amount of current. If EMG responses are produced with only a small amount of current, the screw has likely been placed outside the bony pedicle.
Reactions to Intraoperative Changes in Monitored Responses
Intraoperative changes in evoked responses, such as decreased amplitude, increased latency, or complete loss of the waveform, may result from surgical trespass, such as retractor placement or ischemia, or they may reflect systemic changes, such as changes in anesthetic drug administration, temperature changes, or hypoperfusion. When these changes are detected and considered to be significant, the surgeon or anesthesiologist can make changes to relieve or lessen the insult to the monitored pathway (and presumably surrounding neural structures). Interventions by the anesthesiologist are directed at improving perfusion to the nervous tissue at risk and include increasing arterial blood pressure, especially if induced hypotension is used, or if the patient’s blood pressure has decreased significantly from preoperative values; transfusion, if significant anemia is present; volume expansion; augmentation of cardiac output; and normalization of abnormal arterial blood gas tensions. Changes in evoked potentials after retractor placement during a craniotomy or after compression of the blood supply to the spinal cord from spinal column distraction promptly allow the surgeon and the anesthesiologist to make appropriate changes to the operative procedure and anesthetic management that may prevent or minimize any postoperative neurologic deficit ( Fig. 39.12 ). It is imperative that clear communication occur between all parties in the operating room when significant intraoperative changes in evoked potentials occur. If expected results of an intervention are not observed the underlying hypothesis as to the cause of the changes must be reevaluated. This can only happen when lines of communication are open between all participants.

Tolerance limits for degree of change in evoked response signals or duration of complete loss of waveform before permanent neurologic dysfunction are becoming better defined, and position statements have been issued by the American Society of Neurophysiologic Monitoring ( http://www.asnm.org/page/PositionStatements ). Tolerance limits for degree of change are especially unclear for tcMEPs. Such ambiguity is common among intraoperative monitors. Although we do know that increased frequency and duration of ST segment depression during coronary bypass surgery is associated with an increased risk of perioperative infarction, exact limits for degree and duration of ST segment depression for surgery do not exist, and likely vary significantly from patient to patient. The same problem may be exhibited with neurologic monitors.
Many centers using intraoperative SER monitoring define decreases in amplitude of 50% or more from baseline associated with a less than 10% prolongation in latency as clinically significant SER changes. Uncorrected, such changes are associated in clinical series and in case reports with onset of new postoperative neurologic deficits. As a result, such changes are immediately investigated. In practice, any SER changes directly associated with a surgical event are considered clinically significant, however, even if the magnitude of change is less than just described. Changes in SER that do not progress to complete loss of the waveform are less likely to be associated with a major new postoperative neurologic deficit. Complete loss of the SER waveform intraoperatively without recovery is highly likely to be associated with a major new deficit. If the SER recovers either spontaneously or after intraoperative interventions, the likelihood of neurologic injury depends on the procedure, the duration of the SER loss, and on whether the SER is mainly used to judge integrity of adjacent unmonitored structures. These problems are illustrated in one study of intracranial aneurysm surgery in which SSEPs were monitored to predict/prevent postoperative motor deficits. Loss of the SSEP waveform for less than 15 minutes was not associated with a new permanent neurologic deficit, whereas complete loss of the SSEP for longer periods was increasingly likely to reflect permanent neurologic injury, even if the response recovered completely to its intraoperative baseline during surgery.
Clinical Applications of Neurologic Monitoring
Neurovascular Surgery (Also See Chapters 56 , Chapters 57 )
Extracranial Neurovascular Surgery: Carotid Vascular Surgery (Monitors: Electroencephalogram, Somatosensory-Evoked Potentials, Transcranial Doppler, Cerebral Oximetry)
Carotid vascular surgery typically requires transient interruption of blood flow to the brain through the affected carotid artery. Although the risk of stroke associated with carotid vascular surgery has been declining, the residual risk varies greatly based on the indication for the procedure. It is lowest for asymptomatic patients and highest after a recent revascularization for ischemic stroke.
Electroencephalogram
The use of the EEG as a monitor of the adequacy of hemispheric blood flow during carotid endarterectomy has been established for many years. In a large series of patients undergoing carotid endarterectomy at the Mayo Clinic, the EEG was compared with regional CBF using the Xe washout method. This study validated the EEG as an indicator of the adequacy of regional CBF.
Normal CBF in gray and white matter averages 50 mL/100 g/min. With most anesthetic techniques, the EEG begins to become abnormal when CBF decreases to 20 mL/100 g/min. Cellular survival is not threatened until CBF decreases to 12 mL/100 g/min. The difference in blood flow between when the EEG becomes abnormal and the blood flow at which cellular damage begins to occur provides a rational basis for monitoring the EEG during carotid surgery. In many cases, prompt detection of EEG changes may allow intervention (e.g., shunting, increasing cerebral perfusion pressure) to restore CBF before onset of permanent neurologic damage.
Serious intraoperative reduction in cerebral oxygen supply may result from surgical factors (e.g., carotid cross-clamping) that are usually beyond the anesthesiologist’s control, and from factors that the anesthesiologist can correct. Reduction in CBF produced by hyperventilation, hypotension, or temporary occlusion of major blood vessels may be corrected by reducing ventilation, by restoring normal blood pressure, or, in the case of temporary vessel occlusion, by increasing blood pressure above normal. Because the EEG may readily detect cerebral ischemia, continuous EEG monitoring may be used to evaluate the effectiveness of therapy instituted to correct ischemia.
Should the patient undergoing carotid endarterectomy have EEG monitoring? That question cannot be answered based on available data. EEG monitoring provides information about CBF that would not otherwise be available. The clinician has an opportunity to intervene to increase inadequate blood flow when it occurs. Anecdotally, many clinicians have found such monitoring useful and use it routinely. Population studies do not support routine use, reflecting the fact that strokes in carotid vascular surgery are infrequent and the majority are caused by embolism, which may not be adequately treated by simply increasing CBF.
In a large series of patients undergoing carotid endarterectomy with selective shunting who were monitored with 16-channel unprocessed EEG, no patient awakened with a new neurologic deficit that was not predicted by EEG. Transient, correctable EEG changes were not associated with stroke. Persistent changes were associated with stroke. This study had no comparison group, however, analyzing stroke rate when EEG monitoring was not used during surgery. Conversely, in the North American Symptomatic Carotid Endarterectomy Trial and the European Carotid Surgery Trial, retrospective comparisons of patients receiving EEG monitoring and patients not receiving EEG monitoring failed to show a significant difference in outcome.
Even more difficult to prove is that EEG monitoring is useful when all patients are shunted during carotid clamping. Such monitoring has detected correctable shunt malfunction, and investigators have described hypotension-related EEG changes in patients with critical stenoses and poor collateral circulation. Advocates of selective shunting based on EEG (or other monitoring) criteria claim that inserting a shunt unnecessarily through a region of diseased vessel would surely increase embolization. A multicenter study of 1495 carotid endarterectomies provides some evidence that shunting of patients without evidence of decreased cerebral perfusion increases the incidence of stroke more than sixfold. Although this study and other more recent studies advocate that selective shunting using some form of monitoring of the adequacy of CBF should improve perioperative stroke rate, an analysis by the Cochrane Stroke Group failed to show sufficient evidence to advocate for routine shunting, selective shunting, or even no shunting at all. In addition, this review failed to demonstrate that any type of monitoring for cerebral ischemia was superior to another.
Processed EEG also has been used during carotid vascular surgery. Two issues affect the efficacy and reliability of processed EEG as a monitor for cerebral ischemia. First, what is the minimum number of channels (or areas of the brain) to be monitored? Clinical experience and clinical investigations suggest that four channels (two per side) are the minimum number of channels for adequate sensitivity and specificity. When a limited number of channels were compared with 16-channel EEG monitoring, 100% sensitivity and specificity were obtained using 2 channels per hemisphere, provided that those channels monitored the middle cerebral artery territory. These results were obtained with a frontoparietal channel combined with a frontotemporal channel.
The second issue is the experience level of the observer monitoring the processed EEG. Is a dedicated, experienced technician or electroencephalographer needed? In a study addressing this question, the 16-channel unprocessed EEG monitored by a dedicated technician was compared with a processed EEG reviewed by three anesthesiologists of differing levels of experience with processed EEG. The three anesthesiologists interpreted the tracings without knowledge of the case. They were presented only with the written trace with an indication of the point at which the carotid artery was clamped. In these cases, the most important interpretation pitfall to avoid is the “false-negative” pattern. If the clinician interprets the EEG as showing adequate CBF when in fact it does not, the surgeon may fail to shunt an ischemic patient. A false-positive result may be less of a problem because that patient is not ischemic but is given a shunt anyway. In this case, only the risk of emboli from the “unnecessary” shunt is incurred. The positive predictive value of the anesthesiologist correctly interpreting the trace as unchanged after clamping was 91% to 98%, which indicates that the device can be used by novice interpreters with fair accuracy to determine the presence of cerebral ischemia at the time of carotid occlusion ( Fig. 39.13 ).

Somatosensory-Evoked Potentials
SSEP monitoring also has been used to gauge adequacy of CBF to the cerebral cortex and subcortical pathways during carotid vascular surgery. SSEPs have been found in the laboratory to have a similar but slightly lower threshold for electrical failure compared with the EEG when CBF is reduced. SSEPs are generally intact until the cortical blood flow decreases to less than 15 mL/100 g/min. A recent review (meta-analysis) of shunting during carotid vascular surgery suggests that selective shunting based on SSEP monitoring results in a perioperative stroke rate similar to when EEG monitoring is used as the basis for shunting. However, logic would also suggest that changes in SSEP are unlikely, for example, with ischemia involving the anterior portions of either the frontal or temporal lobe, which could readily be detected by an appropriately placed EEG electrode. There is even less outcome evidence to support the use of SSEPs during carotid surgery than there is for the EEG, but the authors and others have found SSEPs to be useful as a simultaneous monitor with EEG to detect subcortical ischemia.
Transcranial Doppler
TCD monitoring during carotid vascular surgery is based on measurement of two primary parameters—blood flow velocity in the middle cerebral artery and the number of emboli detected in the same artery. The hypothesis justifying the use of this monitoring during carotid vascular surgery has two components: blood flow velocity correlates with CBF, and increasing numbers of emboli increase the likelihood of embolic stroke. Intraoperative use of TCD has not been widely adopted for many reasons. As previously noted, good TCD signals cannot be obtained in up to 20% of individuals who could benefit from monitoring. Also, TCD probe motion during surgery causes major problems with loss of signal or with angle of insonation–induced changes in the relationship between blood flow velocity and blood flow. Nonetheless, multiple series of carotid endarterectomies with TCD monitoring have been reported with good success, quoting a critical blood flow velocity reduction around 50% as indicative of inadequate CBF requiring intervention (shunt or increased blood pressure or both). The relationship between emboli count and stroke is better established. Multiple studies conducted in the preoperative, intraoperative, and postoperative periods indicate that higher emboli counts are associated with higher stroke risk and warrant intervention. TCD is the only monitor able to detect dangerous hyperemia, also known as normal perfusion pressure breakthrough, after removal of a severe, flow-limiting carotid stenosis. Typically, a sustained doubling of flow velocity after unclamping should prompt the anesthesiologist to consider lowering the blood pressure. There are no good outcome data supporting TCD use intraoperatively, but data regarding emboli count and risk of stroke suggest that if technical issues with probe attachment to the patient can be overcome, TCD may be useful as a predictor of impending stroke in the preoperative, postoperative, and perhaps intraoperative periods.
Cerebral Oximetry (Near-Infrared Spectroscopy)
Near-infrared spectroscopy (NIRS) is an attractive monitor during carotid endarterectomy because of its ease of application and lack of training required for interpretation. The hypothesis governing its use is very simple: as oxygen delivery to the brain decreases, oxygen extraction from arterial blood increases, and the oxygen saturation in cerebral venous blood decreases. Multiple case reports and series document the use of cerebral oximetry during neurovascular surgery, but several major questions surrounding use of NIRS during carotid surgery remain unanswered.
The first and most important question is what degree of decrease in oxygen saturation can be tolerated before intervention is necessary. Because most interventions involve some risk (e.g., shunt → emboli, increased BP → myocardial ischemia), the answer to this question is important. The answer does not yet exist, however, and may vary from patient to patient. Two studies in awake patients showed that the saturation value at which any patient would develop symptoms varied from patient to patient, and an absolute value that required shunting could not be determined. Another study showed that cerebral oxygen saturation decreased before the EEG developed changes, and the authors used this observation to claim superiority for NIRS monitoring of the brain during carotid surgery. This finding should not be surprising, however, because function of the brain (in this case, electric function) does not fail until increased extraction of oxygen no longer meets metabolic demands for the tissue. If metabolic demands are being met by increased extraction, it is unclear that intervention is needed.
Finally, a study by Friedell and colleagues compared NIRS with EEG and SSEP monitoring during carotid surgery. In 24 of 323 patients, significant differences were observed between NIRS and monitors of electric function. Seventeen patients showed no changes in electric function with significant decreases in cerebral oxygen saturation. In seven patients, no change in cerebral oxygen saturation occurred despite significant change in the EEG and SSEP. The latter finding may be due to the fact that NIRS and EEG/SSEP monitor different vascular territories. These data in combination with the data from studies in awake patients suggest that use of NIRS alone during carotid vascular surgery may be inappropriate. In addition, an aggregate of studies and case reports available in the literature suggests that there is no clear cutoff value of regional oxygen saturation which would mandate shunt placement or increasing the cerebral perfusion pressure.
Intracranial Neurovascular Surgery (Monitors: Somatosensory-Evoked Potentials, Motor-Evoked Potentials)
Somatosensory-Evoked Potentials
SSEPs have been extensively studied during cerebral aneurysm surgery. During these procedures, the surgical incision and brain retraction diminishes the utility of placing EEG scalp or brain surface electrodes that could detect cerebral ischemia in at-risk cortex. Recording electrodes placed on the surface of the brain have been used successfully, but they are commonly considered “in the way” by neurosurgeons. Thus EEG is typically used only to confirm metabolic suppression during temporary clip placement. Scalp electrodes for SSEP monitoring may be placed more easily, although the recording montage is frequently not the same as that used in an awake patient.
For aneurysms involving the anterior cerebral circulation, SSEP monitoring has an excellent, but not perfect, record for predicting postoperative neurologic function. Most patients without surgically induced SSEP changes during the procedure awaken with an unchanged neurologic examination. Patients with significant SSEP changes that do not revert to normal awaken with a new neurologic deficit. Patients with significant intraoperative SSEP changes that return to normal may show at least a transient postoperative deficit, with the severity and duration of that deficit increasing as the severity of the SSEP change increases. Many authors have reported significant utility of SSEP monitoring in detecting improper aneurysm clip placement (see Fig. 39.12 ) and in guiding intraoperative blood pressure management, particularly in patients already showing, or at significant risk for, vasospasm after subarachnoid hemorrhage. The same success cannot be reported, however, for posterior circulation aneurysms. In these cases, many areas of the cortex and subcortical structures are at risk for damage that cannot be monitored at all by somatosensory pathway function. A significant false-negative monitoring pattern exists for these patients, but changes can still be detected when a surgical insult is sufficiently severe to involve large portions of the brain.
An important concern during aneurysm surgery is injury to perforating vessels near the aneurysm, which risks damage to subcortical pathways in the internal capsule and may account for half the new neurologic deficits immediately postoperatively. Anatomically, the motor pathway runs anterior to the sensory pathway in the internal capsule and is at greater risk if the anterior choroidal artery, lenticulostriate perforator vessels, or even perforating branches of the anterior cerebral artery are injured during dissection and clip placement. Two concerns need to be addressed if tcMEP is to be incorporated successfully in these cases. First, motion caused by stimulation needs to be minimized to not interfere with the surgery. Second and more importantly, stimulus parameters need to be set to limit deep current spread that would activate the corticospinal tract distal to the internal capsule and obscure ischemia of the proximal pathway. The use of longer stimulus trains at intensities close to the motor threshold addresses both concerns and have made tcMEP monitoring a useful adjunct during aneurysm surgery in many centers.
Supratentorial Intracranial Nonvascular Surgery (Monitors: Awake Patient, Electroencephalogram, Somatosensory-Evoked Potentials)
The most comprehensive approach to the problem of functional localization of brain structures that need to be preserved to achieve a good outcome is to perform entire segments of a supratentorial craniotomy in an awake patient, who is undergoing repeated neurologic examinations targeted to assess the eloquent area at risk. Such procedures are typically divided into exposure, mapping, and resection phases, and can be done with the patient entirely awake or awake only during periods when the neurologic examination needs to be assessed. Common to all these approaches is the need for meticulous locoregional anesthesia of the scalp at the craniotomy site and the pin sites of the head holder. A second requirement is a patient who is well informed about the awake parts of the procedure and willing and able to cooperate. Dexmedetomidine, propofol, and remifentanil are the agents most frequently incorporated into the anesthetic regimens for awake craniotomy. Complications of awake craniotomy include nausea and vomiting, respiratory problems, and “tight” brain, but are typically mild and occur in less than 10% of cases in experienced centers. Seizures triggered by cortical stimulation can be stopped by the application of iced saline to the exposed cortex or a small amount of barbiturate or propofol.
Seizure Focus Localization Surgery
Patients with epilepsy who have seizures that generalize from an anatomically distinct focus may benefit greatly from the surgical resection of that seizure focus. Precise localization of a seizure focus is important for achieving the therapeutic objective of seizure control and for minimizing complications from the resection. With sensitive magnetic resonance tomography techniques, neuronavigation, and recordings of typical seizure activity in the awake patient after placement of subdural and depth electrodes, the anatomic location and the appropriate extent of the resection frequently can be determined preoperatively. These developments have diminished the role of intraoperative recordings from the epileptogenic zone using electrocorticography.
Electrocorticography is done by placing a grid of subdural electrodes onto the exposed brain surface and recording spontaneous electric activity. Electrocorticography is constrained by several limitations. The time for such recordings is limited to a few minutes; recordings are limited to interictal discharges, which may not correlate with the epileptogenic focus; and recordings need to be obtained from a brain that is under the effects of general anesthetic agents, which alter the EEG.
To provide good conditions during the recording, the level of anesthesia is lightened (e.g., by use of a strict nitrous oxide–narcotic technique or low concentrations of volatile anesthetic drugs). Provocative techniques, such as hyperventilation or administration of a small dose of methohexital, may be useful to activate the seizure focus. Intraoperative seizure mapping requires the involvement of an expert electroencephalographer familiar with this technique.
Motor Strip Localization
Electrophysiologic monitoring of the somatosensory system in anesthetized patients can provide a simple anatomic guide to the location of the rolandic fissure, which separates the parietal primary sensory and frontal primary motor cortex. The fissure is located by recording cortical SSEPs from a subdural strip electrode that is placed perpendicular to the presumed location of the fissure. The exact location of the fissure is characterized by a reversal in the polarity of the primary cortical response between the electrodes straddling the fissure, as illustrated in the clinical example in Fig. 39.14 . Subsequent placement of the electrode strip onto the primary motor area of the precentral gyrus allows subsequent monitoring of the corticospinal tract through direct cortical stimulation.
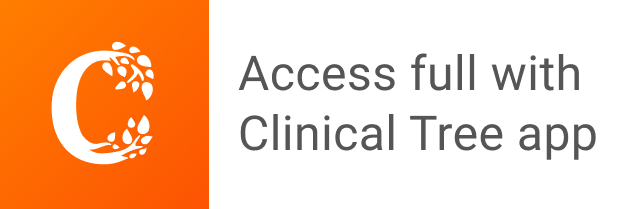