Key Points
- ▪
Critical care of the nervous system is based on control of cerebral and spinal cord physiology and the prevention of secondary insults. This goal, in turn, depends on the comprehensive maintenance and adequacy of physiologic parameters and organ function.
- ▪
Cerebral function is critically dependent on oxygen delivery matching metabolism.
- ▪
Increased intracranial volume beyond the capacity of compensatory mechanisms increases intracranial pressure (ICP) and may diminish perfusion adversely. The resulting cellular energy failure both initiates and propagates edema and inflammation.
- ▪
The resolution of cerebral edema depends on hydrostatic and osmolar forces applied to the blood-brain barrier. Excess perfusion pressure or intravascular hypotonicity worsens edema and must be avoided.
- ▪
Blood-brain barrier disruption varies over time and by pathologic process, and it affects the ability of hypertonic agents to exert a beneficial osmotic effect.
- ▪
Fever is frequently overlooked in the neurocritical care unit, but it significantly affects patient outcomes across a range of pathologic processes.
- ▪
Neurologic monitoring comprises placement of appropriate monitoring devices as well as prompt response and institution of therapy to the changes detected. The goal is to optimize the physiologic environment. Clinical examination of neurologic function remains a crucial part of monitoring and care.
- ▪
The main principle in the treatment of traumatic brain injury involves the control of physiologic parameters such as ICP to achieve an adequate cerebral perfusion pressure. No pharmacological intervention exists to minimize secondary brain damage.
- ▪
After the initial hemorrhage and early brain injury, mortality and morbidity from subarachnoid hemorrhage (SAH) arise from rebleeding and delayed cerebral ischemia. Early treatments of ruptured aneurysms, including medical and endovascular therapies, to improve cerebral perfusion, maintain blood volume, and optimize oxygen delivery have improved outcomes. SAH may be accompanied by significant pulmonary, cardiovascular, or endocrine effects.
- ▪
Successful therapy for ischemic stroke is contingent on a time window of viability. Urgent assessment and rapid treatment are crucial to a good outcome. Endovascular therapy, in conjunction with advances in imaging, has led to significant outcome benefits.
- ▪
Injury to the spinal cord necessitates careful evaluation of respiratory mechanics to assure adequate ventilation.
- ▪
Infections of the central nervous system demand an aggressive approach to volume resuscitation, cerebrospinal fluid sampling, and early empiric antibiotic therapy, similar to treatment for sepsis.
Acknowledgment
The editors and publisher would like to thank the following authors: Michael J. Souter and Arthur M. Lam for their contributions to the prior edition of this work. It has served as the foundation for the current chapter.
Critical care of the central nervous system (CNS) involves collaboration among several disciplines—neurosurgery, anesthesiology, neurology, neuroradiology, and electrophysiology. Each discipline offers unique contributions, which, in partnership, provide optimal care not only to the injured brain but also to the cardiopulmonary, endocrine, gastrointestinal, and renal systems that support cerebral physiology. Integration of the complex goals of this care relies on the physician specializing in neurocritical care. The use of a neurocritical care team, rather than single specialty care, has been associated with reduced in-hospital mortality and the length of stay. Although other specialists can train to be neurointensivists, anesthesiologists with training in neuroanesthesia and critical care are particularly well suited to demonstrate the combination of airway and cardiovascular support skills that, together with an understanding of the physiology and pharmacology of the nervous system, may improve the outcome.
Although the brain has certain preeminence among the organs of the body, it relies on a stable platform of organ function elsewhere to enable homeostatic control and mechanisms of repair and recovery. Injury to the brain is associated with and precipitates a wide spectrum of dysfunction of other organ systems ( Box 84.1 ). Vice versa, brain function can also be perturbed by injury of the other organs. This mutual relationship is best described by the concept of organ crosstalk.
Global | Pyrexia |
Inflammatory activation | |
Cardiovascular | Arrhythmia: bradycardia, tachycardia, atrial fibrillation |
Hypertension | |
Hypotension | |
Left ventricular dysfunction | |
Respiratory | Apnea |
Pneumonia: aspiration, hypostatic, ventilator associated | |
Pulmonary edema | |
Acute respiratory distress syndrome (ARDS) | |
Gastrointestinal | Gastric erosion |
Ileus | |
Constipation | |
Perforation | |
Malabsorption | |
Renal | Dehydration |
Acute renal failure | |
Urinary tract infection | |
Hematologic | Anemia |
Leukocytosis | |
Coagulopathy, disseminated intravascular coagulation | |
Deep venous thrombosis, pulmonary embolism | |
Metabolic or endocrine | Hyponatremia, hypernatremia |
Hypoglycemia, hyperglycemia, | |
Hypokalemia, hyperkalemia | |
Hypomagnesemia | |
Hypophosphatemia | |
Catabolic azotemia | |
Rhabdomyolysis |
Intracranial Physiology and Cerebrovascular Autoregulation
The cerebrovascular circulation is constrained by a rigid perimeter of bone (see also Chapter 11 ). After exhaustion of limited compensatory mechanisms, the bony confines of the skull mandate increasing intracranial pressure (ICP) consequent to increasing intracranial volume. The change in ICP with change in intracranial volume is often referred to as the intracranial compliance curve, but it is more appropriately termed the intracranial elastance curve ( Fig. 84.1 ). The increase in elastance (i.e., changes in ICP as a function of changes in intracranial volume) implies poor compliance, and a small change in volume can lead to an inordinate increase in pressure. These changes in volume are due to the increase in intracranial tissue or fluid content (i.e., blood, interstitial fluid/edema, or cerebrospinal fluid [CSF]). Tissue content is relevant insofar as mass lesions influence intracerebral elastance and accentuate the effects of fluid changes. Small increases in volume can be accommodated by an outflow of CSF from the cranial cavity into the spinal canal, producing an exponential pressure-volume relationship (see Fig. 84.1 ).

The cranial vault is compartmentalized by the falx cerebri and tentorium cerebelli, which creates the possibility of internal pressure gradients ( Fig. 84.2 ). Increased intracranial volume finally leads to herniation of brain tissue through the “apertures” of the compartments. Pressure gradients are initially hydraulically equilibrated, with CSF moving from the ventricular chambers to the extracranial spinal space. Brain herniation may also contribute to impedance of CSF drainage either by occlusion of the foramina of Monro in the case of lateral herniation or by occlusion of the third ventricle and aqueduct by supratentorial herniation through the tentorial gap. This situation typically gives rise to clinical features of midbrain compression. Unilateral pupillary dilation, ipsilateral or even contralateral paralysis (the Kernohan notch phenomenon), and abnormalities of respiration are apparent in the patient. If the herniation continues, it will result in cerebellar descent through the foramen magnum with consequent compression of the brainstem, thus producing bilateral papillary fixation, either tachycardia or bradycardia, and systemic hypertension. Changes in cerebral mass secondary to increase in intracerebral venous blood volume also arise from venous drainage obstruction by compression of the easily susceptible bridging veins draining from cortex to venous sinuses. Once an individual threshold of elastance is crossed, changes in volume exert a mass effect on the draining veins, which act as Starling resistors. This effect decreases drainage, which, in turn, amplifies and prolongs pressure increases. Blood volume increases may be extravascular (i.e., hemorrhage) or intravascular (i.e., accumulation within a predominantly venous capacitance circulation). The other main fluid mass effect is through edema, either cytotoxic or vasogenic. Cytotoxic edema results from hypoxia, with swelling of the intracellular compartment, whereas vasogenic edema in the interstitium usually develops from a breach of the blood-brain-barrier, often in response to high blood pressure.

Changes in the control of cerebral blood volume (CBV) may have significant effects on ICP and, thereby, on cerebral perfusion pressure (CPP). The cerebral vasculature responds to increase in arterial partial pressure of carbon dioxide (PaCO 2 ), decrease of arterial partial pressure of oxygen (PaO 2 ), and reduced mean arterial pressure (MAP) by dynamically altering arteriolar caliber to maintain a constantly matched cerebral blood flow (CBF) sufficient to meet metabolic demands ( Fig. 84.3 ). Carbon dioxide, as with hydrogen, potassium, calcium, nitric oxide, adenosine, and lactate, are among many purported metabolic mediators of flow. A reduction in substrate delivery (i.e., oxygen and nutrients) to less than the threshold necessary to maintain cellular viability induces cell injury with consecutive cytokine and chemokine release. This inflammatory amplification expands the injury, disrupts the blood-brain barrier function, and may directly lead to apoptosis or necrosis. The disruption of the blood-brain barrier creates vasogenic edema and allows serum proteins to cross into brain parenchyma with prolonged effects. This process is more evident in the high metabolically active gray matter than in white matter.

CBF control may be directly compromised by brain injury or by abnormalities of respiration and arterial blood pressure. Consequently, untoward changes in physiologic parameters, including arterial hypotension, hypoxia, hyper- and hypocapnia, hyper- and hypoglycemia, and fever, as well as seizures, produce what is termed a secondary physiologic insult. The primary injury is caused by the direct destruction of tissue during the initial trauma or ischemia, while the area of the secondary injury slowly extends into the intact tissue and induces further harm to the vulnerable brain and worsens outcome. The control of the secondary brain injury is the target of the development of potential neuroprotection protocols.
General Cardiopulmonary Considerations
Arterial hypotension, especially in combination with hypoxemia, is a well-documented source of cerebral morbidity. Therefore, a reduction of cardiac output and CPP have to be avoided to prevent further deterioration of the level of consciousness, which in turn leads to airway compromise and hypercapnia and hypoxia. Hypercapnia, hypoxia, and arterial hypotension lead to autoregulatory cerebral vasodilation, which increases CBV and ICP and further diminishes CPP, perpetuating the vicious circle ( Fig. 84.4 ).

Any deterioration in ventilatory efficacy adversely affects cerebral elastance through carbon dioxide-induced vasodilation and leads to hypoxemia, which can cause direct as well as indirect injury to the brain. Arterial hypoxemia, less than 60 mm Hg, is a significant contributor to secondary insult from vasodilatory ICP effects, in addition to reduced oxygen delivery to the cell. Pulmonary compromise is often seen in neurologic patients with altered mental status due to impaired airway reflexes and repeated aspiration episodes, which culminate in a significant incidence of pneumonia, irrespective of the initiating pathologic process. Another potential mechanism is the release of cytokines from the brain in response to the inflammatory process triggered by an injury. This release may be sufficient to induce acute respiratory distress syndrome (ARDS) and systemic inflammatory response syndrome (SIRS). Conversely, ARDS can also trigger cerebral inflammation by releasing inflammatory mediators like cytokines. The ventilatory treatment for ARDS may create a therapeutic dilemma in the presence of cerebral pathophysiology. However, the “open lung” concept (i.e., small tidal volumes, high frequencies, and high positive end-expiratory pressure [PEEP]) developed to minimize alveolar distention and reduce lung injury appears to be feasible in neurosurgical patients, despite theoretic concerns regarding adverse effects on ICP. The use of PEEP up to 15 cm H 2 O appears to improve brain tissue oxygen pressure and oxygen saturation without negative effects on ICP or CPP. .
Fluids, Electrolytes, and Nutrition
Normally, capillary fluid shift is a function of hydrostatic pressure pushing fluid out and the balance of osmotic forces retaining fluid within :
Jv=Kf([Pc−Pi)−σ[πc−πi])
In extracranial capillaries, these osmotic forces are derived from oncotic pressure because smaller solutes can cross the capillary basement membrane following concentration gradients and only large protein molecules remain to exert their effect. However, in the brain, because of the presence of tight junctions in the endothelium, the intact blood-brain barrier reflects smaller solutes (e.g., sodium and chloride ions), and this relative impermeability renders cerebral capillary fluid shift a function of hydrostatic and total osmolar forces, with the oncotic pressure being responsible for only 1 mOsmol/kg. Therefore, the fluid shift in the cerebral capillaries is most dependent on the osmolar gradient. In the absence of externally administered osmotically active substances (e.g., mannitol), plasma osmolality is 280 to 300 mOsm/kg and is almost entirely determined by sodium concentration.
Plasmaosmolality=[NA]serum×2+glucose/18+BUN/2.8
As a reduction of plasma osmolality of 4 to 5 mOsm/kg increases cerebral edema, hypotonic solutions must not be used in the neurosurgical patient. Table 84.1 shows that the mean measured osmolality is often lower than the calculated osmolarity. Hence, intravascular fluid therapy must be carefully considered under circumstances of cerebral ischemia and inflammation. The brain exerts homeostatic control on metabolic and endocrine activity, and neurologic dysfunction can manifest as untoward changes in fluid and electrolyte balance. Diabetes insipidus is a florid example of this, with polyuria, subsequent hypovolemia, and, if the disorder is left untreated, systemic hypotension. Iatrogenic causes may include the use of osmotic diuretics. Sympathetic denervation stemming from brainstem or spinal cord injury (SCI) may also contribute to reductions in venous return due to increased vasodilation and peripheral venous pooling.
Fluid | Mean Measured Osmolality (mOsm/kg) | Theoretical Osmolarity (mOsm/L) |
---|---|---|
Plasma | 288 [280-300] | 291 |
Isotonic saline 0.9% | 285 [282-286] | 308 |
Ringer´s lactate solution | 257 [257-258] | 276 |
Gelatin 4% | 271 [270-272] | 274 |
Human albumin (4%) | 266 [266-267] | 274.4 |
Nutritional deficits occur often in brain injured critically ill patients, and greater energy and protein deficits are associated with prolonged intensive care unit (ICU) and hospital stays. According to the Brain Trauma Foundation (BTF), early enteral nutrition should be initiated at least by the fifth day and at most by the seventh day post injury. It is possible that an even earlier start at day one after trauma can be beneficial in terms of better control of infection and overall complications. For administration of enteral nutrition, a transgastric jejunal tube is recommended to reduce the incidences of ventilator-associated pneumonia.
Stress-induced hyperglycemia is associated with higher morbidity and mortality in patients with neuronal injury, but tight glycemic control, targeting plasma glucose levels of 80 to 120 mg/dL, increased the risk of hypoglycemia and did not improve outcomes compared to conventional therapy allowing a maximal glucose concentration of 150 mg/dL. Therefore, moderate glycemic control with a target blood glucose level of 110 to 150 mg/dL is advocated.
Temperature Control
Fever occurs with an incidence of up to 70% in brain injured patients. The degree and duration of early hyperthermia are closely correlated with a higher morbidity and mortality after neurologic injury. The temperature threshold that produces progressive thermal injury to the metabolically active brain cells, blood-brain barrier, and vascular endothelium appears to be between 39°C and 40°C. Hyperthermia, an often overlooked insult to the compromised brain, increases oxygen utilization and metabolic stress. Patients in the ICU can have multiple risk factors for hyperthermia such as infection from indwelling catheters (e.g., arterial, venous, CSF) or lung injury. However, in up to one-third of cases, the cause of the fever remains unexplained and often is classified as central fever.
The concept of targeted temperature management in neurocritical care includes therapeutic mild hypothermia, controlled normothermia, and aggressive treatment of fever. Although in head trauma patients, induced mild or moderate hypothermia failed to improve outcome, hypothermia did improve neurologic outcome in neonates with hypoxic-ischemic encephalopathy and in patients after out-of-hospital cardiac arrest. To cool patients, gel pads or intravascular temperature-modulating devices with servo-controls should be used to minimize overshoot. Cold saline infusions are additionally recommended. Core temperature should be monitored continuously using an esophageal temperature probe or a bladder temperature probe. Shivering must be treated promptly with nonsedating interventions (e.g., acetaminophen) instead of narcotic analgesics, sedatives, or paralytics.
Monitoring
The avoidance or correction of secondary brain damage necessitates the use of physiologic monitors to guide individualized therapy. The most important neuromonitoring devices are shown in Fig. 84.5 (see Chapter 39 ). Neuromonitoring does not obviate the need for regular neurologic clinical examination. Similarly, attention should be paid to the basics of volume status evaluation, cardiovascular stability, respiratory care, and metabolic consumption. Monitors (e.g., processed electrical activity, cerebral oxygenation, and ICP) are merely surrogates of actual integrity of brain tissue and cerebral function.

Clinical Examination
Comprehensive neurocritical care encompasses the ability to perform a competent neurologic examination. Reproducible and objective assessment of neurologic function is as important as some of the sophisticated technology mentioned later, with the advantage that it offers better insight into global nervous system function and allows integration of information in an inherently complex dynamic system. One of the most basic yet important examinations is the pupillary light reflex, the unilateral absence of which may indicate midbrain compression from uncal herniation, which is a neurologic emergency. Bilaterally absent pupillary reflexes signify imminent or established cerebellar herniation, but this may be reversible with rapid efficacious treatment.
Clinical scales have been devised for common neurologic settings. The Glasgow Coma Scale (GCS) is a well-known, universally applied scale ( Table 84.2 ). It relies on independent assessment of eye opening, speech, and best motor movement in response to progressive trials of command, voice, and noxious stimuli. Its accuracy is compromised by the use of sedatives/anesthetics. In clinical practice, duration of loss of consciousness, altered consciousness, and posttraumatic amnesia are also considered. The World Federation of Neurologic Surgeons (WFNS) scale is the preferred rating because it uses the more prevalent GCS but with a modifying component for focal deficit ( Table 84.3 ). The Hunt and Hess Scale describes the severity of subarachnoid hemorrhages and is used as an outcome predictor ( Table 84.4 ). Knowing and using these scales are crucial to understanding the terminology and practice of neurocritical care.
Ability | Score |
---|---|
Motor Response | |
Normal | 6 |
Localized to pain (purposeful movement to side of pain) | 5 |
Withdraws to pain | 4 |
Abnormal flexion to pain (an abnormal posture that can include rigidity, clenched fists, legs held straight out, and arms bent inward toward the body with the wrists and fingers bent and held on the chest) | 3 |
Abnormal extension to pain (an abnormal posture that can include rigidity, arms and legs held straight out, toes pointed downward, and head and neck arched backward) | 2 |
None | 1 |
Verbal Response | |
Normal conversation | 5 |
Disoriented conversation | 4 |
Words, but not coherent | 3 |
No words, only sounds | 2 |
None | 1 |
Eye Opening | |
Spontaneous | 4 |
To voice | 3 |
To pain | 2 |
None | 1 |
SUM | 3-15 |
Grade | Clinical Presentation |
---|---|
Grade 1 | GCS score of 15, motor deficit absent |
Grade 2 | GCS score of 13-14, motor deficit absent |
Grade 3 | GCS score of 13-14, motor deficit present |
Grade 4 | GCS score of 7-12, motor deficit absent or present |
Grade 5 | GCS score of 3-6, motor deficit absent or present |
Grade | Clinical Presentation | Survival (%) |
---|---|---|
Grade 1 | Asymptomatic or mild headache | 70 |
Grade 2 | Moderate to severe headache, nuchal rigidity, and no neurologic deficit other than possible cranial nerve palsy | 60 |
Grade 3 | Mild alteration in mental status (confusion, lethargy) and mild focal neurologic deficit | 50 |
Grade 4 | Stupor and/or hemiparesis | 20 |
Grade 5 | Comatose and/or decerebrate rigidity | 10 |
Intracranial Pressure and Cerebral Perfusion Pressure
Intracranial Pressure
Increased ICP after head trauma is an important and well-established indicator of secondary brain injury and is associated with higher mortality and poor long-term outcome, especially when it is refractory to treatment. However, the clinical benefit of ICP monitoring is still under discussion. The indications for ICP monitoring (e.g., severe head trauma and abnormal computed tomography [CT] scan) and the recommended ICP threshold of 22 mm Hg given in the guidelines are more expert- than evidence-based. The sole randomized clinical trial evaluating the potential benefit of ICP monitoring revealed that ICP monitoring does not influence long-term outcome after head trauma. However, ICP-guided therapy in the context of duration of elevated ICP or in combination with information from other neuromonitoring devices may allow for individualized therapy of brain-injured patients and, thereby, improve outcome. For example, the cumulative ICP-time burden with an ICP of 20 mm Hg for longer than 37 minutes diminishes outcome, whereas shorter periods had no adverse effects. Impaired cerebrovascular autoregulation or a CPP below 50 mm Hg reduces the ability to tolerate an elevated ICP.
In clinical practice, two different methods of monitoring ICP are used: microtransducer devices (strain gauge or fiberoptic types) and ventricular catheters. Microtransducer systems are less invasive and easier to place, but they cannot drain CSF and may represent only the compartment pressure where they were placed (see Fig. 84.5 ). The ventricular catheter is still the “gold standard” of measurement, as long as the ventricles are accessible, because they reflect global ICP and allow therapeutic drainage of CSF. However, they have an increased risk of complications, such as bleeding or infection. Currently, noninvasive ICP measurements are not sufficiently reliable and cannot monitor the dynamics continuously, and, thus, are of research interest only.
Cerebral Perfusion Pressure
The difference between MAP (zero reference point at the tragus of the ear) and the ICP describes the CPP. However, many interventions to decrease ICP, such as head elevation or barbiturate coma, suppress MAP and therefore, may also lower CPP. Most guidelines recommend CPP should be kept between 60 and 70 mm Hg, as higher or lower values worsen outcome after head trauma. Recently, the concept of targeting an individualized optimal CPP was introduced, suggesting that an optimal CPP can be calculated for each patient, leading to a more favorable outcome compared with the standard threshold.
Cerebral Blood Flow
A constant, uninterrupted CBF is pivotal for the brain, as it has no storage for energy or oxygen. A decrease in CBF below 20 mL/100 g/min causes functional impairment ( Table 84.5 ). Further reductions in CBF lead to structural damage of brain tissue. Modern imaging techniques such as CT perfusion or positron emission tomography (PET) provide detailed information about cerebral hemodynamics, but cannot clinically be used for continuous monitoring of CBF. Therefore, bedside solutions for continuous CBF measurement are necessary.
CBF (mL/100 g/min) | Result |
---|---|
50 | Normal |
20 | EEG slowing |
15 | Isoelectric EEG |
6-15 | Ischemic penumbra |
<6 | Neuronal death |
Thermal Diffusion Flowmetry
Thermal diffusion flowmetry is an invasive, continuous, and quantitative technique to measure local CBF. The thermal diffusion flowmetry catheter measures the temperature difference between a thermistor, which is heated some degrees above tissue temperature, and a temperature probe. The temperature difference can be converted to an absolute measurement of CBF in mL/100 g/min. The catheter is placed in areas at risk for hypoperfusion and can further help detect intracerebral vasospasm and assess cerebrovascular autoregulation. However, there are still concerns about the validity of using thermal diffusion flowmetry long-term, as monitor dysfunctions secondary to placement errors and missing data during recalibration occur.
Transcranial Doppler Monitoring
Transcranial Doppler monitoring is a noninvasive method that uses the Doppler shift effect to assess flow velocity in insonated cerebral arteries. Flow velocity has a linear relationship to CBF as long as the vessel’s cross-sectional area and the angle of insonation remain constant. This technique can continuously monitor flow velocity within the main components of the circle of Willis through the ophthalmic, temporal, and foramen magnum acoustic windows. It has excellent temporal resolution and can detect inadequate CBF, assess pressure autoregulation and carbon dioxide reactivity, and can help predict outcome in patients with head trauma. Changes in velocity can be used to assess alterations of vascular caliber (e.g., arterial vasospasm) or vascular stenosis. High flow velocities in the middle cerebral artery (MCA) can be caused by vasospasm or hyperemia. This can be distinguished by calculating the ratio between the MCA and the extracranial carotid artery flow velocity (the Lindegaard index).
Cerebrovascular Autoregulation and Vasomotor Reactivity
Monitors of CBF can assess the response of the cerebral vasculature to changes in metabolism and blood pressure (i.e., cerebrovascular autoregulation). Static cerebrovascular autoregulation can be determined with sustained blood pressure manipulation by using either a tilt test or a direct vasopressor, whereas dynamic cerebrovascular autoregulation can be assessed using rapid deflation of thigh cuffs that have been inflated above systolic blood pressure (SBP). For continuous assessment of cerebrovascular autoregulation the pressure reactivity index is an established tool. Using the Pearson correlation coefficient the pressure curves of ICP and arterial blood pressure are correlated, calculating an index between -1 and +1.
Instead of ICP other parameters like the CBF (measured by TCD), the brain tissue PO 2 , or Near Infrared Spectroscopy (NIRS) derived variables can be used to calculate an autoregulatory index to assess the status of the cerebral autoregulation. The presence or lack of autoregulation may guide subsequent treatment and prognosis because the loss of autoregulation is associated with a poor outcome frequently.
Cerebral Oxygenation
To assess the adequacy of cerebral perfusion, measurement of cerebral oxygenation is indicated, because it gives the balance between cerebral oxygen delivery and utilization.
Jugular Venous Oxygen Saturation
The Fick principle may be reversed to examine the venous oxygen saturation which, assuming a constant hematocrit and metabolism, can offer an assessment of the adequacy of CBF and associated oxygen delivery relative to cerebral oxygen consumption:
IfAVDO2=(CMRO2/CBF),thenCaO2−CjvO2=(CMRO2/CBF)
Ignoring the contribution of dissolved oxygen, then:
(SaO2−SjvO2)×Hgb×1.34=(CMRO2/CBF)
In vivo, catheters to measure Sjv O 2 are placed in retrograde fashion through the internal jugular vein to its jugular bulb at the foramen, or even beyond, to the large sinuses ( Fig. 84.6 ). Sjv O 2 can be measured intermittently by sampling venous blood from a catheter or continuously by fiberoptic measurement. The normal range of Sjv O 2 lies between 55% and 75%. Both desaturation (<50%, suggesting inadequate delivery or excess consumption) and abnormally high saturation (>75%, suggesting hyperemia or stroke) have been associated with poor outcomes. The arteriovenous oxygen content difference is possibly a more accurate assessment of the adequacy of flow and predictor of outcome. Jugular venous oximetry is sometimes insensitive to focal changes because it reflects the averaged global cerebral venous oxygen saturation from the confluence of hemispheric venous drainage. Consequently, some authors have suggested using oxygen consumption in combination with the cerebral arteriovenous gradient of lactate to make stoichiometric assessments of aerobic versus anaerobic metabolism. The idea is to base goal-directed therapy on metabolic indices (Sjv O 2 ) rather than on hemodynamic indices (ICP and CPP). However, due to technical problems (e.g., displacement of catheter) and its low sensitivity, the clinical use of jugular venous oximetry has decreased in favor of alternative monitoring methods for brain tissue oxygen monitoring.

Brain Tissue Oxygen Tension
Miniaturized Clark electrodes have been developed and combined with ICP catheters to provide simultaneous measurements of brain tissue oxygen tension (PbO 2 ) and ICP. The PbO 2 should be measured in the area of risk, which contains still viable subcortical white matter. The normal range of PbO 2 lies between 20 and 45 mm Hg. In clinical settings, values less than 15 to 20 mm Hg are considered as cerebral ischemia and below 10 mm Hg as severe ischemia. Several studies have demonstrated a correlation between low PbO 2 and poor outcome after traumatic brain injury (TBI). Therapy guided by combined ICP (<20 mm Hg) and CPP (60-70 mm Hg) and PbO 2 (> 20 mm Hg) was superior to ICP and CPP therapy alone. To increase a low PbO 2 (<20 mm Hg), mechanical ventilator settings should be optimized. A challenge of 100% oxygen for 2 minutes (a temporary method to restore PbO 2 and to verify probe function) can be performed and CPP and ICP, hemoglobin, and sedation should be optimized.
Near-Infrared Spectroscopy
Pulse oximetry for the brain relies on the principle of reflectance spectroscopy in which near-infrared light traverses bone. The light is scattered and reflected in a ratio that is inversely proportional to the concentration of light-absorbing materials in tissue (e.g., hemoglobin and oxyhemoglobin). The surface detector is constructed and calibrated to detect light that has traversed down to the cerebral cortex and back. An adjacent detector is positioned to detect a signal from superficial tissues, and both signals are then used in an algorithm to derive an estimated tissue saturation.
NIRS is a noninvasive approach to continuously monitor a regional critical oxygen supply/demand mismatch. The normal range lies between 60% and 75%, but the lower threshold varies considerably among individual patients. Unfortunately, the sensitivity to detect isolated cerebral hypoperfusion is low, and, therefore, high-quality data supporting the use of NIRS in patients with TBI, SAH, or stroke are missing. In contrast, NIRS is an excellent trend monitor in patients with cerebral hypoperfusion caused by systemic changes, such as during cardiac surgery where a decreased NIRS compared to the awake baseline correlates with a poor outcome.
Brain Metabolism and Biochemistry—Cerebral Microdialysis
Cerebral microdialysis probes can assess the biochemical milieu of the brain. The probes are placed through a burr hole and cycle small volumes of dialysate through the catheter to an extracranial collection system. The tip of the catheter should be placed in at-risk brain tissue, which is most vulnerable to secondary brain damage. A semipermeable membrane (molecular weight cutoff of 20 kDa) is incorporated in the tips of the probes through which various substances can diffuse along their concentration gradient (e.g., lactate, pyruvate, glucose, glycerol, and glutamate) into the dialysate for collection and analysis using a bedside high-pressure liquid chromatography device. As these substances are associated with glucose metabolism, hypoxia/ischemia, and cellular energy failure, the cerebral microdialysis is used to guide individualized intensive care therapy to optimize substrate supply, cerebral perfusion, and oxygen transport. An increased lactate:pyruvate ratio with a low pyruvate concentration in combination with a low-glucose concentration can indicate a profound reduction in energy substrate supply and is associated with poor outcome after brain injury. In contrast, an increased lactate:pyruvate ratio with normal pyruvate and glucose concentrations is an indicator of a nonischemic cause (e.g., mitochondrial dysfunction). Glutamate is a marker of excitotoxic brain damage and glycerol can indicate membrane breakdown of neurons. Recently, probes with a high-molecular-weight cutoff membrane (100 kDa) have been used to detect biomarkers for neuronal damage like S100B or cytokines. Despite promising results, the clinical utility of a therapy guided by cerebral microdialysis is still under discussion.
Neurophysiologic Monitoring
Clinical neurophysiology picks up the electrical activity of the central or peripheral nervous system. It includes electroencephalography (EEG), evoked potentials (EP), and electroneuromyography and is very useful for diagnosis, prognosis, and follow-up of cerebral disorders. However, clinical neurophysiology only evaluates the current functional brain status and cannot predict future complications.
Electroencephalography
The electroencephalogram (EEG) records spontaneous electrical activity of cortical neurons detected by appropriately placed electrodes in a radial and axial array as defined by the 10/20 system, an internationally standardized system of recording. The EEG is a surrogate parameter for functional metabolism of the cortical neurons and the integrity of the brain. The spectrum of component frequencies, together with amplitude and power, can be quantified and analyzed in a variety of fashions, and processed EEG monitors use these to assess the depth of anesthesia. In unconscious patients in the neurocritical care unit, EEG should be continuously used for the detection of nonconvulsive seizures, which can frequently occur and are associated with intracranial hypertension and cerebral metabolic derangements. As the detection of nonconvulsive seizures is difficult, automatic monitoring systems have been developed. Unfortunately, the current automatic detection of seizures is fraught with false negatives and false positives and cannot replace an EEG-trained neurologist.
For prognosis after anoxic brain damage, poor outcome is associated with malignant EEG patterns like burst-suppression, α-coma, and low-voltage delta. In contrast, the persistence of EEG reactivity is an indicator of good outcome. Further development of intracranial electrocorticography, an invasive form of EEG monitoring, might allow the continuous detection of so-called spreading depolarizations, which occur in about half the patients with head trauma and can lead to secondary brain damage.
Evoked Potentials
EPs measure changes in neuronal activity, which are triggered by sensory stimuli. They reflect the passive response of the cerebral cortex or the brainstem to peripheral (acoustic, somatosensory, or visual) or central (transcranial magnetic stimulation) stimuli. In contrast to the EEG, where spontaneous cortical activity is recorded, the EPs monitor the integrity of central and peripheral tracts. The EP signals are quantified according to latency and amplitude. In the absence of peripheral or cervical dysfunction, the bilateral absence of the N 2 0 somatosensory EP response to median-nerve stimulation is the most reliable predictor of non-awakening in post-anoxic coma, whereas the preservation of cognitive evoked potentials (CEP) predicts awakening with a very high probability, irrespective of coma etiology. After TBI, impairment of brainstem activity is the best predictor of bad outcome.
Multimodality Neuromonitoring
Multimodality monitoring uses a combination of parameters to identify, prevent, and treat the mechanisms of secondary brain damage and guide individualized therapy. Various commercial systems are available to process and display multiple data streams. The combination of ICP, cerebrovascular autoregulation, PbO 2 , continuous EEG, and cerebral microdialysis offers the possibility to individualize care decisions in patients with severe brain injury. In the future, the development of computational model interpretation of these complex multimodal data sets will provide summary outputs of patient-specific simulations of brain state to facilitate the interpretation and suggest individual therapies.
Radiographic Imaging
The most important diagnostic modalities for unconscious and brain-injured patients are CT, including CT-angiography, and magnetic resonance imaging (MRI). CT and CT-angiography are very efficient methods, while MRI is time consuming but more sensitive for brainstem lesions as well as for axonal injuries. These imaging techniques are used to diagnose damage after TBI (intracranial masses, cortical contusions, and neuronal/axonal injury), an epidural or subdural hematoma, subarachnoid hemorrhage (SAH), ischemic or hemorrhagic stroke, cerebral vein/sinus thrombosis, or postoperative complications such as brain edema or rebleeding.
It is important to standardize and categorize CT images for meaningful comparison of therapeutic modalities in clinical trials and for assessment of prognosis. For TBI, the novel Stockholm and Helsinki CT scores seem to give a more accurate outcome prediction compared to the Marshall CT classification and the Rotterdam CT score ( Tables 84.6 and 84.7 ). After SAH, the Hijdra sum score seems to be superior to the more commonly used modified Fisher scale in assessing the amount of subarachnoid blood and in predicting the occurrence and severity of cerebral vasospasm. ( Tables 84.8 and 84.9 ). For early assessment of neuronal damage after stroke, the Alberta Stroke Program Early CT Score (ASPECTS) uses signs of early ischemic lesions in regions M1 to M6 and the basal ganglia.
Category | Definition |
---|---|
Diffuse injury I | No visible intracranial pathologic process |
Diffuse injury II | Cisterns are present with midline shift of 0-5 mm and/or lesion densities present; no high- or mixed-density lesions at >25 mL |
Diffuse injury III | Cisterns compressed or absent, with midline shift of 0-5 mm; no high- or mixed-density lesions of >25 mL |
Diffuse injury IV | Midline shift of >5 mm; no high- or mixed-density lesion of >25 mL |
Evacuated mass lesion (V) | Any lesion surgically evacuated |
Nonevacuated mass lesion (VI) | High- or mixed-density lesion of >25 mL; not surgically evacuated |
Criteria | Mild | Moderate | Severe |
---|---|---|---|
Structural imaging | Normal | Normal or abnormal | Normal or abnormal |
Loss of consciousness | < 30 min | 30 min to 24 h | >24 h |
Posttraumatic amnesia | 0-1 day | >1 and <7 days | >7 days |
GCS (best available in 24 h) | 13-15 | 9-12 | 3-8 |
Abbreviated injury scale score: Head | 1-2 | 3 | 4-6 |
Grade | Clinical Presentation |
---|---|
Grade 1 | No blood detected |
Grade 2 | Diffuse deposition of subarachnoid blood, no clots, and no layers of blood <1 mm |
Grade 3 | Localized clots and/or vertical layers of blood ≥1 mm in thickness |
Grade 4 | Diffuse or no subarachnoid blood, but intracerebral or intraventricular clots present |
Grade | Clinical Presentation |
---|---|
Grade 0 | No blood detected |
Grade 1 | Thin subarachnoid blood ∗ , no intraventricular hemorrhage |
Grade 2 | Thin subarachnoid blood, with intraventricular hemorrhage |
Grade 3 | Thick subarachnoid blood, no intraventricular hemorrhage |
Grade 4 | Thick subarachnoid blood, with intraventricular hemorrhage |
∗ Vertical thickness of 1 mm separates thin from thick subarachnoid hemorrhage.
Common Diseases in the Neurocritical Care Unit
Traumatic Brain Injury
Severe TBI is mainly a result of traffic accidents, assaults, falls, and domestic abuse. It is the leading cause of disability and death in young male adults. Poor outcomes after TBI are correlated to older age and are worse after falls. The primary injury, which is defined by the kinetic impact of the trauma, deforms the brain structure. The pattern of this damage covers a wide spectrum from localized contusion to diffusely scattered foci, lacerations, and hematomas varying with the circumstances of the incident and the architecture of the victim’s brain. TBI is classified by whether it is via blunt or penetrating mechanism. Penetrating injury may again have widely varying consequences depending on site, depth, and energy, but is generally fatal if it bilaterally traverses the midbrain. The GCS categorizes TBI into mild, moderate, and severe injury according to the patient’s presenting clinical characteristics and correlates with outcome after TBI (see Table 84.2 ). Additionally, the Abbreviated Injury Scale (AIS) score for the head and neck region classifies TBI by ranking injuries on a six-point scale based on mortality risk, with “1” indicating minor injury and “6” indicating a nonsurvivable injury. Both scores are comparable in predicting short-term mortality after severe TBI.
The management of TBI in the ICU should include comprehensive examination and assessment using such principles as outlined in the Advanced Trauma and Life Support protocol because occult injuries can escape initial screening.
Pathophysiology
As the brain has no tolerance for hypoxia, TBI patients need immediate treatment according to current guidelines. Unfortunately, the extent of the primary injury cannot be influenced by therapy and, therefore, prevention (e.g., by helmets or airbags) is the only way to avoid damage. The primary brain damage triggers a cascade of pathophysiologic changes, which then lead to secondary brain damage. During the first post-traumatic days, the aim of treatment is to minimize the growth of the secondary damage as much as possible in order to rescue salvageable brain tissue. Pathologic processes such as elevated ICP (due to edema, hemorrhage, obstruction of CSF flow), diminution of arterial inflow, and consequent reduction in CPP with resulting tissue hypoxia and loss of cerebrovascular autoregulation increase secondary brain damage. Inflammation, massive release of excitatory neurotransmitters, apoptotic cell death, high lactic acid concentrations due to anaerobic glycolysis, depleted adenosine triphosphate (ATP)-stores, increased intracellular Ca 2+ concentrations, generation of free radicals and proteolysis are some of the identified contributors to secondary brain damage. Unfortunately, despite intense research and characterization of all these mechanisms, no drug has been identified that can improve outcome after TBI in randomized, prospective clinical trials. This is most likely related to the complex pathophysiology of brain damage, the heterogeneous patterns of damage, and various preexisting patient diseases. TBI also leads to disturbances in other systems such as: sympathetic discharge of the autonomic nervous system, inflammatory responses, endocrine dysfunctions, electrolyte imbalances, cardiovascular and respiratory disturbances, and coagulation impairments. These systemic effects must be monitored and immediately treated, as they also contribute to secondary brain damage.
Traumatic SAH (tSAH) occurs in up to 60% of admissions for TBI and influences outcome after TBI. Approximately 20% of patients with tSAH may also develop vasospasm, which causes secondary ischemic insult.
Treatment
All therapeutic strategies focus on optimization of the delivery of oxygen and glucose to the brain cells. These strategies include maintaining adequate CPP, controlling ICP, and optimizing oxygenation. Therefore, in the critical care setting, the management of TBI patients should follow established protocols with close monitoring of parameters, including CPP, ICP, and oxygenation status. Clinical assessments like continuous measurement of arterial blood pressure, heart rate, and pulse oximetry in combination with monitoring volume status, urine output, and GCS have to be performed.
Cerebral Perfusion Pressure
CPP results from the difference of MAP minus ICP and should be kept between 60 and 70 mm Hg. Aggressive attempts to maintain CPP above 70 mm Hg with fluids and vasopressors should be avoided, as this treatment increases the risk of respiratory failure. Arterial hypotension with a SBP below 90 mm Hg is strongly correlated to poor outcome and has to be avoided in the management of TBI patients. There exists a smooth U-shaped relationship between systolic or MAP and outcome without any evidence of an abrupt threshold effect. Therefore, while current recommendations are to maintain SBP above 100 mm Hg for patients 50 to 69 years old or at or above 110 mm Hg for patients 15 to 49 or over 70 years old, new data possibly suggest that an optimal SBP of 135 mm Hg should be the target.
Intracranial Pressure
Elevated ICP above 22 mm Hg is associated with increased mortality and should be treated with measures according to the checklist in Box 84.2 . This includes optimizing patient position, osmotherapy, deep sedation with barbiturates or propofol, and ventricular drains. Hyperventilation reduces CBV and ICP due to its vasoconstrictive effect, but at the same time hyperventilation leads to a mismatch between oxygen delivery and oxygen consumption. Therefore, hyperventilation is only a temporizing measure until other ICP-lowering measures are available. Decompressive craniectomy has been a strategy to lower ICP, but unfortunately this intervention increases the number of patients surviving in a vegetative state or with severe brain damage. Prophylactic mild hypothermia was also a promising intervention to reduce ICP; however, in prospective multicenter studies, this therapy was not superior to normothermia. ICP control with high-dose steroids was shown to have an adverse effect on mortality and morbidity in a multicenter study of more than 10,000 patients with brain injury. Therefore, steroids are not recommended for control of ICP after TBI.
- 1.
Keep physiological variables in normal range (normotension, normocapnia, normoxia, normothermia, normoglycemia, normovolemia)
- 2.
Head position (30-degree elevation); avoid rotation of the head
- 3.
CPP 60-70 mm Hg; massive fluid-therapy or high doses of vasoconstrictors should be avoided
- 4.
Normocapnia (PaCO 2 = 35-40 mm Hg); if ICP >20-25 mm Hg, then induce short-term hyperventilation (PaCO 2 = 30-35 mm Hg)
- 5.
Provide adequate sedation
- 6.
CSF drainage if ventricles are still detectable
- 7.
Consider mannitol or hypertonic saline
- 8.
Consider barbiturate therapy (under EEG monitoring)
- 9.
Fever control
CPP , Cerebral perfusion pressure; CSF , cerebral spinal fluid; EEG , electroencephalogram; ICP , increases intracranial pressure; PaCO 2 , arterial partial pressure of carbon dioxide.
Oxygenation and Ventilation
Patients with GCS scores of 8 or less should be intubated and ventilated with a target of PaO 2 above 80 mm Hg. If PEEP is necessary, levels up to 15 cm H 2 O have been shown to increase brain tissue oxygen pressure and oxygen saturation without increase in ICP or decrease in CPP.
Sedation
TBI patients should be sedated with drugs with a short context-sensitive half-life like propofol to facilitate daily examination of their neurologic condition. Care should be taken to screen patients for propofol infusion syndrome, which can occur when high-dose propofol is used over several days. Most of the barbiturates and benzodiazepines have a longer half-life and are, therefore, less suitable. Low-dose inhalational anesthetic can also be used. At higher concentrations, volatile anesthetics possess a direct vasodilatory effect, which increases CBV and, in turn, ICP. Ketamine was contraindicated in TBI patients due to perceived risks of causing intracranial hypertension, but in intubated and ventilated patients, ketamine has no adverse effect on ICP. Ketamine has several favorable effects like reduced need of supplementary vasopressors and narcotics, activation of bowel movement, and bronchodilation. Narcotics, like sufentanil, fentanyl, and remifentanil, have no negative effects on ICP as long as MAP is maintained. Muscle relaxants can be used in TBI patients, with the potential exception of succinylcholine, which possibly increases ICP. Nitrous oxide (N 2 O) and etomidate should not be used in patients after severe TBI.
Additional Interventions
In TBI patients, enteral nutrition is recommended to attain basal caloric replacement between the fifth and the seventh day after TBI. Enteral feeding using a transgastric jejunal tube should be started as soon as possible. Early tracheotomy can facilitate the weaning of the patients and reduce mechanical ventilation days, but there is no evidence that it reduces mortality or the rate of nosocomial pneumonia. Up to 25% of patients with isolated TBI develop deep vein thrombosis (DVT) with the risk of pulmonary embolism. Low-molecular-weight heparin or low-dose unfractionated heparin should be used in combination with mechanical prophylaxis, despite the increased risk of intracranial hemorrhage expansion. The Parkland Protocol, which stratifies patients into different risk groups for spontaneous progression of hemorrhage, can help assess the optimal timing for the start of DVT prophylaxis. The incidence of early posttraumatic seizures (during the first week after TBI) can be reduced by phenytoin. As these early posttraumatic seizures do not influence outcome, this prevention is not obligatory. Late posttraumatic seizures are not susceptible to prophylactic interventions.
Spinal Cord Injury
In up to 5% of all major trauma cases, the spinal cord is injured and about 14% of these patients suffer from an unstable spine injury. The American Spinal Injury Association (ASIA) has classified SCI into five categories, where ASIA A represents a complete impairment and ASIA E represents normal sensation and motor function ( Table 84.10 ). Surgical decompression of the spinal cord should be performed within 24 hours after SCI and is associated with improved neurologic outcome.
Grade | Clinical Presentation |
---|---|
Grade A | Complete. No sensory or motor function is preserved in the sacral segments S4-S5 |
Grade B | Incomplete. Sensory but not motor function is preserved below the neurological level and includes the sacral segments S4-S5 |
Grade C | Incomplete. Motor function is preserved below the neurological level, and more than half of key muscles below the neurological level have a muscle grade less than 3 (Grades 0-2). |
Grade D | Incomplete. Motor function is preserved below the neurological level, and at least half of key muscles below the neurological level have a muscle grade greater than or equal to 3 |
Grade E | Normal. Sensory and motor functions are normal. |
Damage to the sympathetic outflow to the heart and vasculature after SCI contributes to systemic hypotension and bradycardia due to unopposed vagal tone, commonly referred to as neurogenic shock. Injuries above T7 have an 85% risk of serious cardiovascular instability. To avoid secondary injury after SCI, the MAP should be kept above 85 to 90 mm Hg during the first 7 days after injury using fluid and vasopressor therapy. Fluid therapy should be monitored by cardiac output monitoring devices and hypotonic solutions like dextrose 5% in water, Ringer´s lactate, and 0.45% sodium chloride should be avoided, as they worsen cord edema. Vasopressors should be inotropic, chronotropic, and vasoconstrictive like α 1 – and β 1 -agonists (dopamine, norepinephrine). Dobutamine has generally no indication because of its β 2 -receptor activation and its vasodilatory effects. In cases with life-threatening bradycardia, a cardiac pacemaker placement can be considered.
The need for ventilatory support depends on the level of injury. The muscles contributing to respiration are the diaphragm (phrenic nerve, C 3-5), the intercostal muscles (thoracic nerves), and the accessory muscles, including the sternocleidomastoid (cranial nerve 11) and scalene muscles (cervical plexus). Respiratory failure secondary to abdominal and intercostal muscle paralysis can still occur even with injuries below C5 because these muscles significantly increase the efficiency of diaphragmatic contractions. The traumatic sympathectomy also leads to intestinal atony with subsequent abdominal distention, which further deteriorates the efficacy of the already compromised diaphragm. With complete cervical SCI, acute respiratory failure is common, secondary to the sudden loss of functional residual capacity and the inability of the sternocleidomastoid muscle to stabilize the chest wall. As hypoxia leads to poor outcome after SCI, patients who develop respiratory insufficiency should be intubated as soon as possible. Weaning should be started early and if it is complicated, the patient should receive a tracheostomy to reduce mechanical ventilation days, decrease the need for sedation, and facilitate pulmonary toilet.
Lower limb blood flow is reduced with increased arterial and venous pooling. This is associated with a higher incidence of thromboembolic disease, and early administration of venous thromboembolism prophylaxis within 72 hours after injury is recommended. Inferior vena cava filters are not suitable for prophylaxis.
Many drugs have been tested for their potential neuroprotective effects; however, high-dose methylprednisolone, monosialotetrahexosylganglioside (GM-1), riluzole, fibroblast growth factor, minocycline, or magnesium have not improved outcome after SCI in prospective, randomized, multicenter studies. Early mild hypothermia after SCI showed some promising results in clinical studies; however, they have to be validated by prospective, randomized, multicenter studies.
Cerebrovascular Disease
Cerebrovascular diseases are the leading cause of death and disability worldwide. Although clinical presentations and outcomes are highly variable, initial management often requires critical care resuscitation. Ischemic strokes are the most common (80%-90%) of stroke subtypes and are increasingly a larger proportion of post-procedural ICU management. Hemorrhagic strokes account for the remaining 10% to 20% of strokes, but are often the most medically complex and have the longest length of stay and highest morbidity and mortality in the ICU. The following sections will provide an overview of the most common critical care management issues for SAH, ischemic stroke, and intracerebral hemorrhage (ICH).
Subarachnoid Hemorrhage
SAH is a neurologic emergency that requires critical care management. Accounting for less than 5% of all strokes worldwide, SAH is associated with severe morbidity and mortality. Most cases of SAH are aneurysmal in origin (aSAH). The incidence of aSAH per 100,000 person years is variable by ethnicity and geography—the lowest incidence being in China (2), followed by Central and South America (4.2), United States (8-15), and the highest being in Finland and Japan (19-23). Women have a greater risk than men by a factor of 1.24, with a higher incidence among blacks and Hispanics compared to Caucasians. Most aneurysms occur between ages 40 and 60 years, with a mean age of 55 years. Risk factors for aSAH include cigarette smoking, hypertension, heavy alcohol abuse, use of sympathomimetic agents, family history of SAH, and prior history of aSAH. Between 10% and 15% of patients will die before hospitalization, and 25% will die within the first 48 hours. The initial hemorrhage is usually the primary cause of death, followed by rebleeding. Case fatality rates had continued to decline in recent decades from 50% to 33% with the advent of endovascular treatment, microsurgical techniques, and improved ICU management.
Early Brain Injury
The degree of neurologic abnormalities and the amount of bleeding are the strongest predictors of clinical outcome and complications after SAH. The Hunt and Hess grading system and World Federation of Neurological Surgeons Scale (WFNSS) are the most widely used (see Tables 84.3 and 84.4 ) with higher WFNSS and Hunt and Hess grades associated with worse clinical outcomes. In the first 72 hours after the acute bleed, multiple mechanisms such as transient global ischemia, elevated intracranial pressures, and toxicity from SAH have been implicated in early brain injury. Subsequent effects on CBF, microcirculatory changes, cerebral edema, and sympathetic response can lead to both neurologic and systemic complications. Acute management of blood pressure and oxygenation are critical in this period along with the prevention of early complications such as rebleeding, acute hydrocephalus, and elevated ICPs. Ideally, patients should be managed in high-volume facilities with dedicated neurocritical care units.
Rebleeding
Rebleeding is a serious complication that significantly worsens outcomes and increases mortality as high as 70%. The risk of rebleeding is the highest within the first 24 hours (4%-15%) after the initial bleed, remains elevated for the next 2 to 4 weeks (1%-2% per day), then eventually decreases to 2% to 4% annually after the first 6 months. To reduce the risk of rebleeding, guidelines recommend securing the ruptured aneurysm by surgical clipping or endovascular coiling as soon as possible and ideally within the first 24 hours after symptom onset. Since the publication of the International Subarachnoid Aneurysm Trial, endovascular coiling is the recommended treatment when the location, size, and morphology of aneurysm are favorable. Some aneurysms may be more suitable for surgical clipping. There should not be any delay in securing a ruptured aneurysm, regardless of the treatment modality chosen. When surgical clipping or endovascular coiling are delayed, short-term (less than 72 hours) treatment with antifibrinolytic agents (aminocaproic acid or tranexamic acid) is recommended. In addition, seizure prophylaxis can be considered until the aneurysm is treated along with blood pressure control to normotensive goals.
Acute Hydrocephalus
Acute hydrocephalus develops in 25% to 30% of patients after SAH, and emergency ventriculostomy may be lifesaving. Patients with hydrocephalus can develop progressive deterioration leading to stupor and coma as well as more subtle clinical signs of gaze palsy, pupillary dysfunction, and cognitive slowing. Head CT showing ventricular dilation can be diagnostic and can facilitate early ventriculostomy placement. Hydrocephalus after SAH can be caused by obstruction of CSF outflow by blood products or by impaired CSF absorption by arachnoid granulations. Most patients should have ventriculostomy drains removed when they no longer require external drainage and ICPs have stabilized. Some patients will develop delayed hydrocephalus (3-21 days after the onset), and 20% of patients will need ventriculoperitoneal (VP) shunt placement for chronic hydrocephalus.
Neurogenic Cardiac and Pulmonary Disturbances
Cardiac and pulmonary disturbances are common after SAH and are thought to be secondary to a surge in catecholamine levels and sympathetic tone. Significant autonomic nervous system disturbance after SAH can result in changes in the electrocardiogram and lead to myocardial dysfunction. Transient ECG abnormalities range from sinus tachycardia, peaked T-waves, T-wave inversions, and QT prolongation to ST segment depression or elevation. Electrocardiographic changes are frequent but do not appear to relate to outcome.
Cardiac dysfunction, however, can have a significant impact on management after SAH. Abnormal echocardiography has been described in up to 8% of SAH patients. Poor grade SAH patients can develop sudden hypoxemia and cardiogenic shock with or without pulmonary edema. This phenomenon of neurogenic stunned myocardium can cause severe limitation of cardiac contractility, which is reversible and associated with a good recovery profile. Patients with SAH have echocardiogram changes similar to takotsubo cardiomyopathy, which is also an acute transient dysfunction resulting from sympathetic overactivity. Adrenoreceptor polymorphism has been implicated in myocardial stunning. Different receptor genotypes have an increased sensitivity to catecholamines and are associated with a 3- to 4.8-fold increased risk of cardiac injury and dysfunction.
Troponins are frequently elevated in about 30% of patients after acute SAH. High serum levels are associated with increased hemorrhage, hemodynamic fluctuations, and worse outcomes. Release of troponin can represent cardiac ischemia in patients with atherosclerotic heart disease or a “myocardial leak” from neurogenic injury.
Cardiac dysfunction may complicate and limit the active treatment options for delayed cerebral ischemia (DCI) and increased ICP. A typical strategy for cardiovascular management is given in Box 84.3 based on comprehensive consensus guidelines.
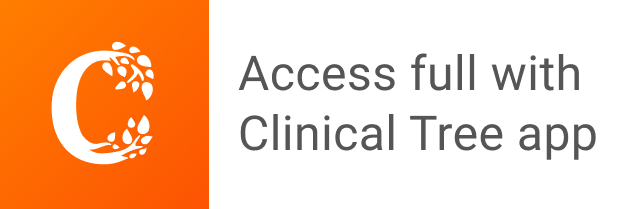