Chapter 32
Musculoskeletal System Anatomy, Physiology, Pathophysiology, and Anesthesia Management
Somatic musculature is broadly classified into three compartments—skeletal, cardiac, or smooth—based on the muscles’ anatomic and functional roles. Force generated by all these muscle types depends on the transient elevation of intracellular calcium (Ca2+) and activation of actin and myosin filaments. Skeletal muscle is under voluntary control and is striated in appearance. Smooth muscle is found in most internal organs (except the heart), is under involuntary autonomic control, and is nonstriated. Cardiac muscle is striated in appearance and under control of an intrinsic pacemaker modulated by the autonomic nervous system.1 The focus of this chapter is skeletal muscle, its function, and its neurologic control.
Skeletal muscle tissue includes muscles of the tongue and the soft palate, the extrinsic eye muscles, the muscles that move the scalp, all muscles attached to the skeleton, and the muscles in the pharynx and the upper third of the esophagus. Some skeletal muscles, such as those of the lips and the anus, serve as sphincters. Skeletal muscle is innervated by myelinated efferent motor nerve fibers called alpha (α) motor neurons. These fast-conducting somatic fibers arise from cell bodies located in the ventral horn of the spinal cord gray matter (Figure 32-1).
At the muscle, each motor nerve divides into branches that enter the muscle and end on individual muscle cells called muscle fibers. A single motor neuron and all the muscle fibers it innervates are collectively called a motor unit (Figure 32-2). When a motor nerve fires, all the fibers within a single motor unit contract simultaneously.
FIGURE 32-2 A motor unit. One motor neuron can synapse with several muscle fibers, which contract as a unit.
Motor units exhibit considerable variability, and each unit usually contains between 100 and 200 muscle fibers. However, the motor unit may contain as few as two muscle fibers for fine, delicate movements or as many as a thousand for coarse movements.1,2
Overview of Neuromuscular Transmission
The process of muscle contraction begins when the electrical activity of a presynaptic motor neuron communicates across a junctional cleft, or synaptic gap, to postsynaptic skeletal muscle fibers. The specialized conduction area, or synapse, where the axon of a motor neuron ends on a skeletal muscle fiber is called the neuromuscular junction, or myoneural junction. Each skeletal muscle fiber usually has only one neuromuscular junction, a notable exception being extraocular muscles that have multiple innervations per cell. The mediator substance that chemically transduces the axon’s electrical message across the synaptic gap to the muscle is the neurotransmitter acetylcholine (ACh).1–3
Muscle contraction develops when the propagated action potential of the presynaptic motor neuron induces expulsion of the chemical mediator ACh into the junctional cleft. ACh binds to specialized receptors on the postsynaptic muscle membrane. If released from the axon nerve ending in sufficient quantity, ACh-receptor (AChR) occupation induces a transient change in the electrical property of the skeletal muscle membrane, and an action potential and muscle contraction follow.2
Neuromuscular Junction
Motor nerve endings develop in intimate and precise proximity to skeletal muscle fibers. The motor axon terminal is separated from the muscle cell it innervates by a synaptic gap of only 20 to 30 nm.2 The demyelinated distal portion of the motor axon is essentially surrounded by a terminal Schwann cell that reinforces the connection of nerve and muscle.4 This anatomic alliance increases the likelihood for prompt receptor activation after transmitter release.2,4,5
The synaptic gap is contiguous with the extracellular fluid, which provides a route for drugs or toxins to gain access to the neuromuscular junction. Botulinum toxin, for example, gains access to the junction through the extracellular fluid and produces its depressive neuromuscular effects by inhibiting ACh release from the nerve ending.2,6,7
Both sides of the neuromuscular junction, the presynaptic motor axon and the postsynaptic muscle cell, serve specialized functions. As it nears the neuromuscular junction, the motor nerve axon loses its myelin sheath and divides into many smaller nerve fibers, which terminate as end-feet.
The motor nerve end-foot is distinct from the rest of the nerve. It is rich in mitochondria and the materials and support structures necessary for the synthesis, storage, mobilization, and release of the neurotransmitter ACh. Small, clear vesicles or granules are particularly numerous in the part of the nerve ending closest to the junctional gap, approximately 300,000 normally in a single end plate.2 Each of these vesicles contains a small packet, or quantum, of ACh molecules. The ACh vesicles concentrate along the junctional surface of the nerve end-feet in areas called “active zones.”6,8
At the neuromuscular junction, each motor nerve ending closely approximates with a thickened and highly convoluted portion of the postsynaptic membrane called the motor end plate. The motor end plate is physically and functionally demarcated from the surrounding muscle membrane. The many membrane convolutions at the end plate are known as junctional folds. Both the primary and secondary folds expand the surface area of the postsynaptic membrane.2,4 ACh receptors are concentrated near the shoulders of the junctional folds, lying near the ACh release sites. The close approximation of ACh release site and target receptor site ensures little transmitter waste and direct coupling of nerve signal and muscle response.4,9 Figure 32-3 summarizes the anatomy and dynamic function of the neuromuscular junction.
Acetylcholine Release
Physiologic transmission of the nerve message to the muscle begins with a Ca2+-dependent mechanism for ACh release from the nerve terminal. When a nerve impulse arrives at a motor nerve ending, the action potential causes a transient increase in Ca2+ conductance across the nerve membrane by activating voltage-dependent Ca2+ channels. Both “fast” and “slow” Ca2+ channels appear to open, but it is primarily the fast (“N-type” and “P/Q-type”) Ca2+ channels that are involved in depolarization-induced transmitter release.6,9 Calcium enters the nerve terminal, flowing down its electrochemical gradient. The influx of Ca2+ causes ACh vesicles to fuse with the nerve plasma membrane and then expel their content into the synaptic cleft.4,6,10 The amount of ACh released is influenced by the amount of Ca2+ that enters the nerve terminal during nerve stimulation. The more Ca2+ that enters the nerve terminal, the greater the amount of ACh released.
About 150 to 200 ACh vesicles, or quanta, are released with each nerve impulse. Each quantum in turn contains about 10,000 molecules of the neurotransmitter.2,11 This amount of ACh and the normal abundance of postjunctional receptors at each neuromuscular junction readily ensure muscle activation. A considerable safety margin exists in the synaptic transmission. With each nerve impulse, excess ACh is released, and excess ACh receptors are available for occupation.9,11–13
Small concentrations of other divalent cations can compete with and limit Ca2+ influx into the nerve ending, decreasing ACh release and impairing neuromuscular transmission. When administered intravenously, magnesium sulfate, for example, can interfere with Ca2+ influx and produce muscle weakness by inhibiting ACh release.3,12
Certain antibiotics, particularly the aminoglycosides, inhibit ACh release from the nerve terminal and can enhance neuromuscular blockade when administered concomitant with clinical dosages of neuromuscular blocking agents.3,11,12
Calcium channel–blocking drugs used for the treatment of dysrhythmias and hypertension block Ca2+ conductance through so-called “slow” (“L-type”) channels. Their primary action is on the slow Ca2+ channels of the heart and blood vessels, but they can inhibit prejunctional Ca2+ influx. The large safety margin inherent in normal neuromuscular transmission obscures any clinically detectable effect these drugs may have on neuromuscular transmission. However, with disorders associated with impaired neuromuscular transmission, such as myasthenic syndrome, the Ca2+ channel blocker’s prejunctional attenuation of ACh release may be unmasked, and neuromuscular transmission may be further weakened.10,12,14
Acetylcholine Synthesis
Choline is obtained locally by a Na+-linked uptake into the cholinergic nerve ending. Acetyl CoA is synthesized from pyruvate in neuronal mitochondria. Mitochondria and other metabolic machinery used to synthesize ACh are abundant in the nerve ending (Figure 32-4).
About 80% of the newly synthesized ACh is stored within synaptic vesicles in the nerve terminal, positioned for release. Each nerve ending contains more than 300,000 of these vesicles. The remainder of the ACh is stored in a nonvesicular axoplasmic reserve.2,4
The ACh vesicles are released through exocytosis in response to action potential stimulation, but only a small fraction of the available vesicles is used to send each signal.2,4
Postjunctional End Plate
There is a distinction between the postjunctional cation channels of the muscle end plate and other cation channels of nerve and muscle membranes. Motor end-plate cation channels are ligand-gated, that is, they are opened or closed by the action of a chemical. Cation channels of nerve axons, on the other hand, are voltage-gated by electrical changes in the membrane.5
The binding of ACh molecules to postsynaptic receptor proteins causes a transient increase in conductance in ligand (ACh)-gated cation channels at the postjunctional motor endplate. The cation flow at the end plate produces a net inward Na+ current and a net outward K+ current. The previously polarized end-plate membrane (resting membrane potential of approximately −90 mV) becomes transiently “depolarized.” The resulting postjunctional membrane voltage change is called the end-plate potential (EPP).5 The EPP does not begin until 0.5 millisecond after the arrival of the action potential at the presynaptic nerve ending. This synaptic delay arises from the relatively slow liberation and diffusion of ACh across the junctional cleft.2,5
Perijunctional Area
The postjunctional end-plate membrane does not fire action potentials. After it is depolarized by ACh-receptor occupation, the current sink created by the local EPP depolarizes the adjacent muscle membrane. If the depolarizing input is great enough and reaches threshold potential, action potentials are fired from either side of the end plate in both directions along the muscle fiber (Figure 32-5).5
The transition zone where the potential developed at the end plate is converted to an action potential is called the perijunctional area. A demarcation exists between the chemically sensitive AChR channels of the end plate and the chemically insensitive but electrically sensitive Na+ channels in the perijunctional area of the muscle membrane. The membrane in the perijunctional area is rich in Na+ channels, and this feature enhances its capacity to respond to an EPP and transform it to an action potential.
Acetylcholine Receptor
Postjunctional neuromuscular ACh receptors have been extensively purified and studied in detail.4,15 An estimated 50 million tightly packed nicotinic AChR sites are at each neuromuscular junction.
In fetal muscle, the ACh nicotinic receptor is a protein composed of five polypeptide subunits: two identical alpha (α) subunits, a beta (β) subunit, a gamma (γ) subunit, and a delta (δ) subunit. Figure 32-6 shows the receptor subunits organized in a pentagonal array around a central ion channel. Without ACh occupation, the central channel is closed; when open, small cations (Na+ and K+) are allowed to pass through the channel down their electrochemical gradients.5
In adults, the AChR protein structure is similar, except that the fetal γ subunit is replaced by an epsilon (ε) subunit.3,16 The change produces an adult cholinergic postjunctional receptor that has an increased cation conductance and a shortened open time.
The ACh receptors are synthesized in the muscle cells and then incorporated into the end-plate membrane as integral membrane proteins. The extracellular or junctional face of the receptor protrudes from the surface of the end-plate membrane, whereas the cytoplasmic surface of the receptor is more flush to the plasma membrane surface (Figure 32-7).
Activation of the postjunctional AChR and opening of the cation channel requires simultaneous ACh occupation at each of the two α-receptor subunits. The binding of two ACh molecules causes a conformational change in the α polypeptides, and the protein conformational change causes the central ion channel to open.2 If only one α subunit site is occupied by the agonist, the channel remains closed. As described earlier, the open channel increases the conductance to positively charged ions, particularly Na+, an effect that produces the net depolarizing potential, the EPP. When even one ACh molecule leaves an α subunit, the channel snaps shut and the current stops.
The α subunits are the sites of competition between the cholinergic agonist ACh and receptor antagonists, such as nondepolarizing neuromuscular blocking agents. The outcome of the competition, neuromuscular transmission or neuromuscular blockade, depends on the concentration of ACh and the relative concentration and binding properties of the antagonist involved.3,12 Nondepolarizing muscle relaxants produce neuromuscular blockade, in part because they bind to one or both α subunit sites and, in so doing, prevent ACh from binding to both sites and opening the channel.
Prejunctional Receptors
Cholinergic receptors are also present at the prejunctional motor nerve ending. It is postulated that in addition to mediating nerve transmission at postjunctional receptor sites, ACh also acts on prejunctional receptors to enhance transmitter mobilization and release. Prejunctional cholinergic receptor occupation may transform the ACh pool from a reserve store to a readily releasable store so that transmitter output can keep pace with transmitter demand.10
All the nondepolarizing muscle relaxants used in anesthesia practice compete with ACh for postjunctional cholinergic receptor sites to produce neuromuscular blockade. Receptor antagonist effects at prejunctional receptors may augment nondepolarizing blockade by diminishing ACh output as well. Herein also lies an explanation for the fade that is observed with neuromuscular blockade monitoring of nondepolarizing muscle relaxers. Fade of tetanic and train-of-four stimulation may reflect the blockade of prejunctional ACh receptors by the muscle relaxant and failure of ACh release to keep pace with rapid stimulation.12
Acetylcholinesterase
As noted earlier, the combination of ACh with its muscle end-plate receptor causes a transitory depolarization of the end plate. The EPP is short lived because soon after binding, ACh is rapidly destroyed by hydrolysis, and its depolarizing action halts.5 Paradoxically, the rapid destruction and removal of ACh from the junctional cleft is critical for continued muscle contractile response. The ACh molecule must be off the muscle end-plate receptor for the perijunctional muscle membrane to repolarize, or “reset,” in anticipation of further activation.
The hydrolysis of ACh to choline and acetate is rapid and efficient. Most ACh is destroyed within a few milliseconds after it is released into the junctional cleft.2 The enzyme acetylcholinesterase (AChE), also known as true or tissue cholinesterase, catalyzes the hydrolysis.
Without AChE, the concentration of ACh would become extremely high in the junctional cleft. Under these circumstances, ACh would maintain the muscle end plate in a state of persistent depolarization as ligand-gated cation channels remained open, yet the muscle itself would be paralyzed.12 The reason for this seemingly illogical behavior (ACh-receptor occupation, end-plate depolarization, yet no muscle contraction) is that in the face of persistent end-plate depolarization, the Na+ channels of the perijunctional muscle membrane do not reactivate or reset; these voltage-gated ion channels remain closed, impeding further muscle membrane depolarization. Thus, even with persistent end-plate depolarization, muscle contraction is prevented, and clinical weakness follows. A cyclic muscle membrane depolarization/repolarization sequence is necessary for normal muscle contraction to occur.
The mechanism of depolarizing muscle relaxants can, at least in part, be explained by a similar mechanism. Depolarizing muscle relaxants, such as succinylcholine, activate the muscle end plate in a manner similar to that of ACh, but they have a more protracted end-plate depolarizing response because they are less rapidly metabolized. AChR occupation by a depolarizing muscle relaxant causes a prolonged depolarization of the end plate, prohibits activation of perijunctional channels, and produces a depolarizing block.3,17
Extrajunctional Receptors
In utero, before muscle innervation occurs, the muscle cells of a fetus synthesize extrajunctional receptors. These fetal receptors (γAChR) as well as receptors made up of five α7 subunits are inserted over the entire length of the muscle cell. As the fetal neuromuscular junction develops, increasing motor nerve activity appears to have a trophic effect in restricting the ACh receptors specifically to the neuromuscular junction.12 By the age of 2 years, the nerve-muscle contact is fully mature and active, and the extrajunctional receptors disappear from the peripheral part of the muscle. If neural activity is reduced or abolished and the neural trophic influence is lost, the muscle resorts to fetal-like synthesis of γAChR and α7 receptors.6,7,12,18
Several situations, including stroke, spinal cord transection, thermal trauma, direct muscle damage, and prolonged immobility, have been associated with the accelerated spread of both γAChR and α7 receptors from the end-plate region to large areas of the skeletal muscle membrane.6,7,18,19 These so-called denervation injuries result in an abnormal excitability of the muscle and an increase in muscle sensitivity to ACh, a condition that is called denervation hypersensitivity.1,20 The extrajunctional receptors may develop within 48 hours after diminution of nerve activity. Eventually, the number of aberrant receptors per muscle fiber may increase 5- to 32-fold.20 These receptors disappear and muscle sensitivity returns to normal if neural input is reestablished. Extrajunctional and end-plate cholinergic receptors are similar in many ways, but an important distinction pertinent to anesthesia practice is their differing response to receptor agonists and antagonists.
Clinically, extrajunctional receptors demonstrate a resistance to nondepolarizing muscle relaxants. Hence, larger doses of nondepolarizing relaxants may be necessary to induce neuromuscular blockade—for example, in an immobilized limb or in parts of the body affected by a stroke.3,4,7,12 Monitoring a nondepolarizing neuromuscular block with a peripheral nerve stimulator in a paretic limb may result in an underestimation of the magnitude of neuromuscular blockade in nonparetic muscles.
Conversely, extrajunctional receptors are more easily activated by agonists (e.g., ACh, succinylcholine) than junctional receptors. Moreover, each extrajunctional channel stays open about four times longer than junctional receptors, allowing more ions to flow (primarily Na+ into the muscle cell and K+ out) in response to agonist-induced depolarization.3
The clinical significance of denervation injuries and the proliferation of extrajunctional receptors becomes evident with the administration of succinylcholine, which can produce alarmingly high levels of plasma K+ in these patients.12,21 Succinylcholine-induced hyperkalemia reflects the extensive proliferation of extrajunctional receptors along the entire muscle membrane and their prolonged and exaggerated depolarization response to agonists. Succinylcholine stimulates the aberrant cholinergic receptors and triggers a protracted opening of the cation channels, allowing excess Na+ movement into the cell and excess K+ movement out, down their respective gradients.
Dangerous levels of succinylcholine-induced hyperkalemia have been observed within 4 days of denervation injury with doses of succinylcholine as low as 20 mg.21 The pronounced release of K+ in response to succinylcholine cannot be circumvented by the prior administration of nonparalyzing doses of nondepolarizing muscle relaxants.
Muscle Physiology
Skeletal muscle constitutes the greatest mass of somatic musculature. Skeletal muscle is composed of bundles of multinucleated, long, cylindrical cells. Muscle fibers typically extend the entire length of a muscle.2 Because their length is much greater than their width, these cells are called muscle fibers. Each muscle fiber is a single cell surrounded by an electrically polarized cell membrane called the sarcolemma. The sarcolemma separates the extracellular space from the myoplasm, the muscle-fiber intracellular space. Dystrophin is a large protein that is located on the intracellular side of the sarcolemma and serves to stabilize the muscle membrane during contraction.1
Individual skeletal muscle cells are parallel to the muscle body and have no anatomic or functional bridges between them. The parallel arrangement helps maximize shortening capacity and velocity. The cells function independently so that the force of contraction of the total muscle is equal to the sum of individual fibers. This contrasts with smooth and cardiac muscle, in which the muscle cells are interdependent and are mechanically coupled to adjacent cells.1,2
Bundles of cylindrical filaments called myofibrils run along the axis of the muscle fiber. Each skeletal muscle fiber contains several hundred to several thousand myofibrils. The myofibrils are composed of contractile proteins that impart a striking, repetitive, light-and-dark banding pattern along the entire fiber length. The repeating unit, called a sarcomere, is the basic contractile unit of skeletal muscle. The alternating light-and-dark banding pattern is responsible for the classification called striated muscle. Cardiac muscle is also classified as striated muscle because it too has the repetitive pattern of light and dark bands.1,2 The arrangement of the muscle fibers, myofibrils, and sarcomeres is shown in Figure 32-8.
Structure of the Contractile Apparatus
The thick filaments, which are composed primarily of the protein myosin, are in the central region of the sarcomere in a dark-colored area termed the A band. A densely staining M line in the middle of the A band contains proteins that link the thick filaments. The thin filaments are about half the diameter of the thick filaments and are composed of the proteins actin, troponin, and tropomyosin. The less dense areas of the sarcomere, which contain only thin filaments, are referred to as I bands. Thin filaments, connected to Z lines or Z disks, partially interdigitate with the thick filaments in the relaxed muscle. With muscle contraction, force is generated by interaction and further overlap of thick and thin filaments. Two adjacent Z lines delimit each repeating sarcomere unit. The diagram of the sarcomere units in Figure 32-9 may merit careful study.
Cross sections of the myofibril reveal that each thick filament is surrounded by a hexagonal arrangement of thin filaments. The myosin and actin filaments are arranged to slide over one another, overlap, and create shortening of the sarcomere and the muscle during contraction2 (Figure 32-10).
Thin Filament
The three major proteins that compose the thin filament—actin, tropomyosin, and troponin—each play a different role in the contractile process. Each thin filament includes two beadlike chains of polymerized actin twisted into a helix. Tropomyosin molecules are located along the groove between the two actin chains. Each rod-shaped tropomyosin molecule covers about six or seven individual actin proteins. The most important protein in the regulation of the contractile process is troponin.1 As depicted in Figure 32-11, troponin is attached intermittently to tropomyosin molecules.
Thick Filament
The tail regions of several hundred myosin molecules aggregate to form one thick filament. The globular heads project out laterally from the thick filament at regular intervals toward the thin filaments surrounding it. In the relaxed muscle, the myosin heads are oriented toward but not attached to the thin filaments. The thick filament’s globular projections are termed cross-bridges because they can link the thick and thin filaments. The cross-bridges in each half of the sarcomere are oriented in opposite directions away from the midpoint of the filament, which is important for their functional role in sarcomere shortening and muscle contraction.1 The cross-bridge components are arranged as shown in Figure 32-12.
The myosin head and tail have a hingelike attachment, permitting a certain degree of movement. When muscle contraction is activated and myosin and actin link, the ability of the myosin head to swivel enables the attached actin filaments to slide over the thick myosin filaments (Figure 32-13).
Cross-Bridge Interaction and Cycling
The sarcoplasmic reticulum is an irregular, closed membrane structure that weaves throughout the myoplasm of the muscle cell and contains large amounts of Ca2+. The sarcoplasmic reticular membrane is active in sequestering Ca2+ by way of numerous high-affinity Ca2+ active-transport carriers in its membrane. These pumps maintain a high sarcoplasmic reticular store of Ca2+ and a very low resting myoplasmic Ca2+ concentration.1,9
The transit of an action potential along the sarcolemma and into the T-tubule system is detected by intracellular voltage sensors that trigger Ca2+ efflux from the sarcoplasmic reticular stores. The major Ca2+ release channels located on the sarcoplasmic reticulum are called ryanodine receptors. These channels are commonly called “receptors” because they bind the alkaloid ryanodine.1 In response to action potential stimulation, the myoplasmic Ca2+ concentration rises several-fold from a resting value of less than 0.1 mmol. The overall effect is the discharge of Ca2+ from the sarcoplasmic reticulum into the myoplasm by the transit of an action potential into the muscle cell.9
The myosin filaments’ heads contain not only an actin-binding site but also a catalytic adenosine triphosphatase (ATPase) site that hydrolyzes the breakdown of ATP to adenosine diphosphate (ADP) and phosphate. ATP is essential for the sliding of the filaments and for muscle contraction. The energy yielded by the ATP hydrolysis is harnessed to tilt the myosin heads, in a “cocked” spring position. When the myosin heads bind to the actin site, the heads use the energy stored in the myosin heads to drag the actin filaments towards the center of the sarcomere. The pull of the actin filaments accentuates the overlap of the thick and thin filaments, causing shortening of the sarcomere and culminating in muscle contraction (Figure 32-14). Cross-bridge cycling and the associated movement of actin is called the sliding filament theory.
The overall process by which depolarization of the muscle fiber causes Ca2+ release from the sarcoplasmic reticulum into the myoplasm to cause cross-bridge cycling and muscle contraction is called excitation-contraction coupling.9
Box 32-1 summarizes the excitation-contraction coupling events. Box 32-2 summarizes the events leading to skeletal muscle relaxation.
Grading Contractile Force
The other mechanism by which skeletal muscle tension is graded is by varying the frequency of the action potential discharge to the muscle. A single action potential invariably liberates sufficient Ca2+ ions to activate skeletal muscle contraction. However, the Ca2+ ions are rapidly transported back into the sarcoplasmic reticulum before the muscle has time to develop maximal tension. The brief contraction that results from transit of a single action potential is called a twitch.2
Unlike cardiac muscle, skeletal muscle does not have a refractory period. Because of this property, rapidly repeated electrical impulses can cause summation of contractions and greatly increase the muscle tension. Repetitive action potentials maintain a high Ca2+ concentration in the myoplasm. The greater the myoplasmic Ca2+ concentration, the more cross-bridge sites are exposed, and the stronger the force of the contraction. Maximal and sustained muscle tension without relaxation—produced by the fusion and summation of successive twitch responses—is called tetanus.9
Figure 32-15 shows the time course and relationship between a single action potential, the myoplasmic Ca2+ rise, and the resulting twitch response. The action potential lasts about 2 to 4 milliseconds. The twitch begins about 2 milliseconds after the start of the muscle membrane depolarization. The duration of the twitch varies with the type of muscle stimulated.
Slow Versus Fast Muscle
Skeletal muscle fibers are classified as type I (“slow fibers”) or type II (“fast fibers”), based on different myosin isoenzymes that distinguish the two types. The two fiber types differ in their metabolic demands, their myosin ATPase activity, and their cross-bridge cycling rates. Muscles usually contain a mixture of both types of fibers, but one type often predominates.1,22,23
Slow (type I) muscle fibers are adapted for sustained movements, such as maintaining posture, and are resistant to fatigue. They have slow twitch durations, depend on oxidative metabolism for energy, have extensive blood supplies, and have rich concentrations of mitochondria.1,22,23 An example of a type I muscle is the soleus muscle of the leg, an important muscle used for maintaining posture and walking. Type I muscle is called “red” muscle because its high myoglobin content imparts a dark, rubrous color.
Fast (type II) muscle fibers usually predominate in “white” muscle and are primarily concerned with rapid or powerful movement. They have short twitch durations, depend on glycolytic pathways for energy, and are easily fatigued.1,23 Muscles specialized for fine, skilled movement, such as extraocular muscles, and some muscles of the hand are in this category.
The diaphragm is engaged in continuous rhythmic activity and so the muscle fibers must be resistant to fatigue. Infants have a greater tendency toward respiratory failure in part because at birth only 25% of their diaphragm is composed of type I fatigue-resistant fibers, compared with 55% in the adult. Before 27 weeks’ gestational age, type I slow fibers make up less than 10% of the total diaphragm muscle content.22
Energy Sources for Skeletal Muscle
Muscle contraction requires a continual supply of energy at a rate proportionate to its energy consumption. The energy consumed by skeletal muscle is used for (1) cross-bridge cycling during muscle contraction, (2) sarcoplasmic reticular resequestration of Ca2+ during muscle relaxation, (3) rephosphorylation of creatine to replenish creatine phosphate energy stores, (4) activation of the Na+/K+ pump to restore proper membrane polarization, and (5) resynthesis of muscle glycogen.2
The amount of ATP present in the muscles is sufficient to sustain maximal muscle power for only about 3 seconds. For this reason, actively contracting muscles must continually form new ATP. There are three metabolic systems that provide a continuous supply of ATP in muscle cells: (1) the phosphocreatine-creatine system, (2) the glycogen-lactic acid system, and (3) the aerobic system.23
Phosphocreatine—Creatine System
The energy-rich phosphate bonds in muscle phosphocreatine (also called creatine phosphate) supply a limited amount of energy to produce ATP in skeletal muscle. The hydrolysis of creatine phosphate to creatine and phosphate is an extremely rapid reaction that releases large amounts of energy for the conversion of ADP to ATP. Creatine phosphate provides a stored source of energy that is used for short bursts of muscle power or for use at the very beginning of muscle contraction while other, more sustainable, energy-regenerating systems are being turned on.2
Glycogen—Lactic Acid System
Skeletal muscle stores glucose as energy-rich glycogen. With brief, intense muscle exertion, muscles meet their energy demands from the breakdown of glycogen to glucose (glycogenolysis) and the metabolism of glucose to pyruvate and lactate (glycolysis). Glycolysis occurs without the consumption of oxygen in the cytoplasm of the muscle cell. The energy released from the breakdown of glucose to pyruvate or lactate is used to convert ADP to ATP for short to moderate periods of muscle contraction.23
Aerobic System
When oxygen is plentiful, the skeletal muscle takes up products of the glycolytic pathway and other substrates, such as amino acids, fatty acids, or ketone bodies, and efficiently oxidizes them to CO2 and water in the muscle fiber mitochondria. This energy-yielding process is called oxidative metabolism. The oxidation of products of the glycolytic pathway and free fatty acids is a slow process, but it is usually sufficient to meet the continual but more modest energy demands of the most frequently used skeletal muscle. The aerobic system is the primary source of energy for muscle cells during prolonged exercise and for steady-state requirements.23
Musculoskeletal Pathophysiology and Anesthesia
Myasthenia Gravis
Incidence
Myasthenia gravis is the most prevalent neuromuscular disorder. With variability depending on country and time of study, a recent incidence rate ranged from 3 to 30 people in every 1,000,000 with myasthenia gravis.24 In individuals younger than 50 years, the ratio of women to men with the disease is 3 to 2; however, in those older than 50 years, the disease is equally distributed between the sexes. Myasthenia gravis can begin spontaneously at any age, but it occurs most often in women between the ages of 30 and 40 years and in men between the ages of 50 and 60 years.11,25 The onset may be abrupt or insidious, and the course is fluctuating, marked by periods of exacerbation and remission.26 Spontaneous remissions that do occur sometimes persist for years.
Pathophysiology
Electron microscopic examination of the neuromuscular junction of the patient with myasthenia gravis shows a decrease in the number of functional postsynaptic ACh receptors (Figure 32-16). The AChR lesion appears to be caused by immune-mediated destruction, blockage, or inactivation. The prejunctional ACh pool is normal.11
Myasthenia gravis is an autoimmune disease with two distinct forms. In the most prevalent form, circulating antibodies react with myoneural AChR proteins, leading to varying degrees of dysfunction. Anti-AChR antibodies (AChR-Ab) are found in the sera of 80% to 85% of patients with myasthenia gravis, but the antibody level does not necessarily correlate with the severity of the disease.27–30
A subset of AChR-Ab-seronegative myasthenia gravis patients have muscle-specific kinase (MuSK) antibodies. MuSK-MG patients have more bulbar muscle involvement and are at increased risk for aspiration. Up to 50% of these patients are likely to experience myasthenia crisis requiring mechanical ventilation.27–31 Serum concentration of MuSK-MG correlates with severity of symptoms.27 Evidence from case series have demonstrated increased prevalence in people living closer to the equator.28,32
The initiating stimulus for the production of IgE antibodies against AChR or MuSK is unclear. The antibodies are T cell–dependent. The thymus gland seems to play a central role in the pathogenesis.11,28,29 The thymus produces autoantibodies that cross-react to antigens in the neuromuscular junction.28,33,34 The thymus is hyperplastic in approximately 65% and abnormal in about 75%. Thymomas, thymic tumors, are present in an additional 10%. Thymectomy is a viable treatment option for such patients.11,28 Thymectomy improves symptoms, but is not curative, suggesting that antibodies are also produced elsewhere.34
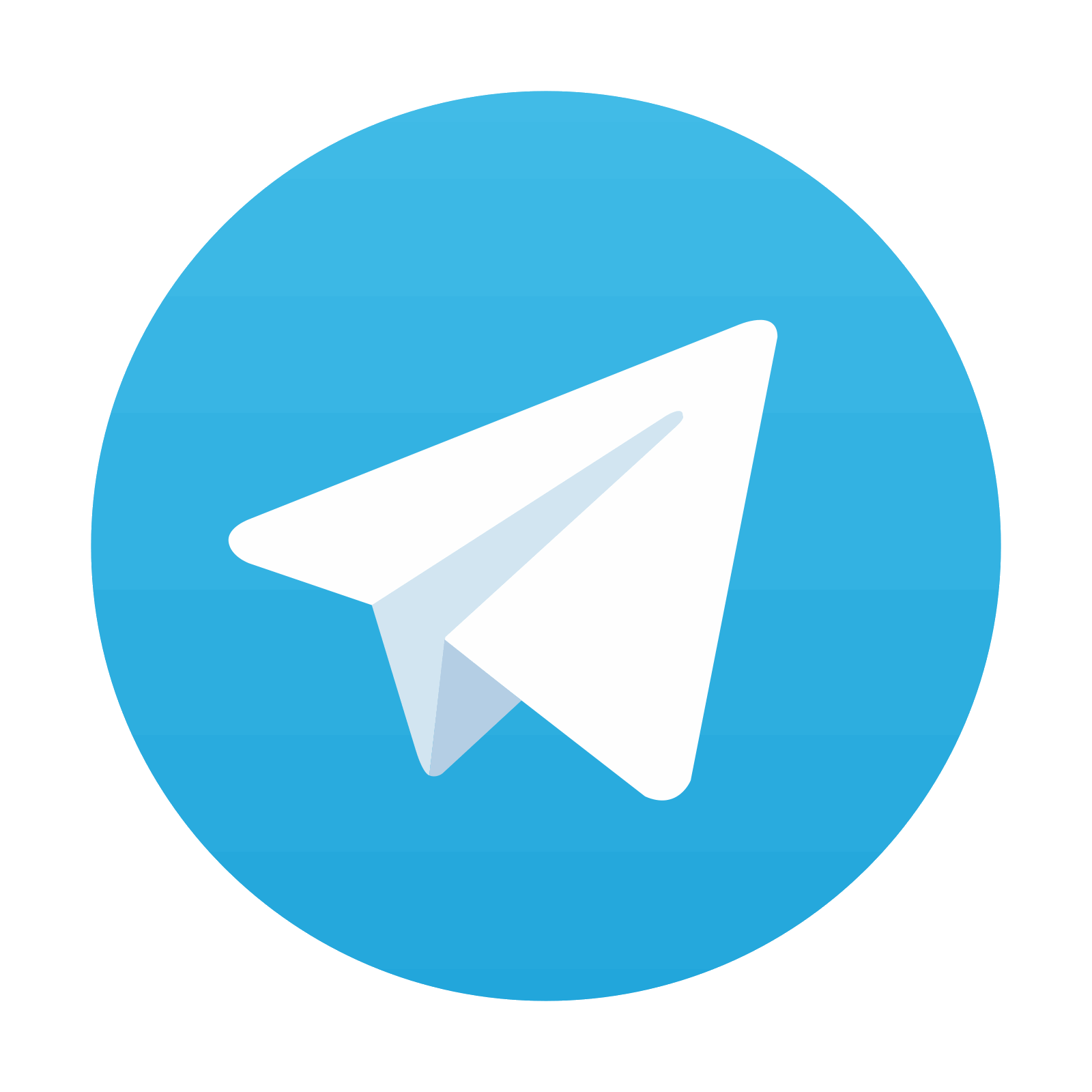
Stay updated, free articles. Join our Telegram channel
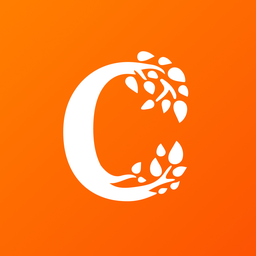
Full access? Get Clinical Tree
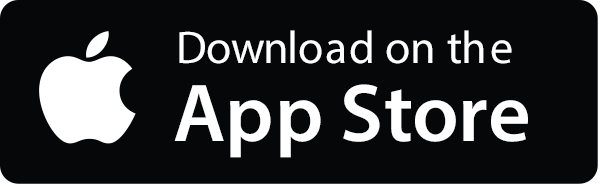
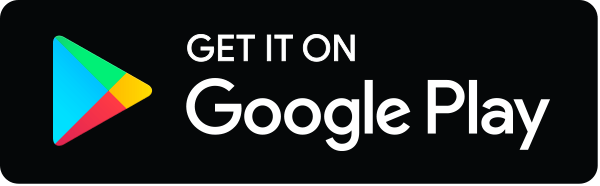