Fig. 46.1
Blood flow velocity measured by TCD during carotid endarterectomy. Preinduction (a). Internal and external carotid arteries clamped (b). Significant decrease in BFV occurs with loss of pulsatility. With unclamping (c), significant emboli are observed in the middle cerebral artery. Postischemic hyperemia (d) is detected (From Eng et al. [104]; with permission)
Vasospasm
TCD has been reported to be an early indicator of the presence of vasospasm prior to the onset of a clinically evident ischemic deficit. These observations are based on the physical principle that, with narrowing of a basal cerebral artery, blood flow velocity would increase, even as flow decreased. Thus, TCD has found a routine place in many neuro-ICUs as a noninvasive screening tool for the occurrence of vasospasm [110–113]. However, subsequent studies, perhaps related to increased use of nimodipine, have shown that increased velocity in subarachnoid hemorrhage (SAH ) patients is associated with hyperemia more often than it is associated with vasospasm [114]. In addition, TCD can miss distal spasm. Thus, TCD, although still possibly of value as a rough screening tool, has insufficient sensitivity and specificity to be the sole criterion to make important therapeutic decisions in SAH . It still may be used as a trend once it is established that a patient clearly is in vasospasm or is hyperemic. Alternatively, it may be used to assess the extent of vasodilatory cerebrovascular reserve, and thus provide indirect information regarding how close a vasospastic condition is to producing clinically evident symptoms [115–117]. It is unclear whether this approach can differentiate hyperemia versus vasospasm .
Cerebrovascular Reserve
Analogous to similar measures with XeCT CBF , determination of the extent of vascular reserve can also be done with TCD and provides a semiquantitative indicator of the severity of injury to a given vascular bed. Reactivity assessment is done by manipulation of either perfusion pressure or carbon dioxide. For a given change in mean arterial pressure, within the normal autoregulatory range, there should be no change in blood flow velocity. A change in flow velocity indicates abnormal autoregulation. Moreover, this has been further developed such that the degree to which changes in blood flow velocity match changes in blood pressure has been developed into a TCD-based autoregulation index, Mx77.
Carbon dioxide reactivity can be assessed by increasing inspired CO2 or by increasing tissue CO2 via administration of acetazolamide. Blood flow velocity normally should increase 3–4 % per mm Hg increase in PaCO2. Failure of brain to do so implies lack of cerebrovascular reserve such that the brain may not tolerate minor perturbations in O2 delivery. A CO2 reactivity index can be defined with CO2 inhalation [118]:
Normal CO2 reactivity index is 1.78 ± 0.48 (SD). In patients with cerebrovascular disease, this index was found to vary from 0.15 to 2.6 [119]. Failure of the brain to demonstrate a normal increase in blood flow velocity with a CO2 increase implies the presence of maximal vasodilation.

Intracranial Pressure
Increases in intracranial pressure into the 20- to 30-mm Hg range, although of epidemiological significance, have not been shown to have substantial physiologic significance in terms of a dangerous decrement in CBF (although there may be important negative effects due to semiocclusive narrowing of bridging veins) [39, 120, 121]. As ICP increases, cerebral vasodilation occurs in a compensatory fashion and the actual CBF tends to not be in the ischemic range at lower ICPs [122]. However, with further ICP increases approaching diastolic arterial pressure [123] and infringing on the critical closing pressure of the cerebral microvasculature [47, 48], flow, which ordinarily is continuous throughout the cardiac cycle, becomes discontinuous, decreasing to zero during diastole. This is consistent with the theoretical conclusions of Giulioni et al. [122] wherein they suggest, based on considerations of intracranial elastance and vasomotor tone, that systolic increase and diastolic decrease in BFV should occur when ICP reaches the “breakpoint” value. They suggest that the Gosling Pulsatility Index should be a useful indicator of high ICP. ICP in such situations of zero diastolic flow theoretically should be in the 40- to 60-mmHg range [47, 48]. Indeed, an ICP of 48 mmHg is the average level at which patients progressing to brain death have been observed initially to sustain a high ICP and a systolic spike pattern with the oscillating pattern developing at 62.5 mmHg [124]. Hassler et al. [123] clearly showed the relationship between ICP and phasic blood pressure and how TCD waveform can reflect ICP encroachment on diastolic flow when ICP exceeds diastolic pressure. This pattern is shown in Fig. 46.1b.
As intracranial pressure increases or cerebral perfusion pressure decreases, the character of the TCD waveform thus changes, becoming more “spiky” in appearance with increased pulsatility [122, 123] (Fig. 46.1). This is the basis for the definition of the pulsatility index ([systolic flow velocity-end-diastolic velocity]/[mean diastolic velocity]) [125]. As diastolic perfusion is increasingly compromised, the cerebral circulation takes on more characteristics of the higher resistance peripheral circulation with lower diastolic flow velocity. Ultimately, diastolic flow velocity is zero as cerebral perfusion becomes discontinuous. Based on observations such as this, TCD may be useful to make general inferences about cerebral perfusion pressure and, specifically, to know if ICP exceeds diastolic blood pressure [126, 127].
ICP assessment with TCD is based predominantly on retrospective reports, having not yet been assessed prospectively in a large number of patients to determine whether TCD could be used to reliably and noninvasively determine the CPP in a given patient. Nonetheless, using TCD to make inferences about ICP is gaining attention in cases where invasive ICP monitoring is contraindicated due to coagulopathy [128, 129]. Given the difficulty making direct ICP measurements, TCD waveform analysis should also be of value in cases of posterior fossa hypertension, although this remains unvalidated .
Brain Death
As cerebral perfusion pressure progressively decreases to sustained levels associated with no CBF (Fig. 46.1), brain death ensues. With TCD this manifests as diastolic reversal of flow presumably due to blood “bouncing” backward off an edematous brain as demonstrated by Hassler et al. [123]. Thus, TCD may be useful as a screening tool for brain death. However, it is important to consider the diastolic blood pressure when making such assessments. A strikingly similar TCD waveform can be generated in a totally sentient patient with aortic insufficiency and diastolic pressure low enough to be in the range of normal intracranial pressure. Notably, a normal TCD waveform should be a good way to rule out brain death. This can be remarkably useful in some operative circumstances.
Vessel Patency
Occasionally, a patient may be admitted to the neuro-ICU after having had a procedure to the middle cerebral or internal carotid arteries. In such cases, TCD has been used during or after surgery to confirm continued patency of the blood vessel [130]. As clinical symptoms may not occur until the vessel is completely occluded or may not occur at all, especially with confounding effects of an anesthetic, TCD may be used to indicate when flow in the monitored vessel is changed in an unwanted manner.
Emboli
Emboli can be readily detected with TCD. This is most commonly a relevant question during cardiac or carotid surgery (Fig. 46.2) [131]. In the ICU, TCD can help determine the adequacy of anticoagulation in patients with artificial cardiac valves or patients with tenuous proximal vascular patency [132, 133]. However, it has been observed that emboli are very common in some situations, without obvious neurological sequelae. Thus, determination that a given frequency of emboli in a given patient warrants major changes in therapy is presently a matter of clinical judgment.
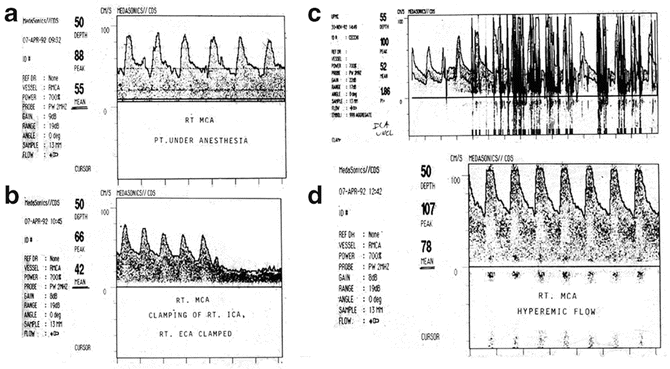
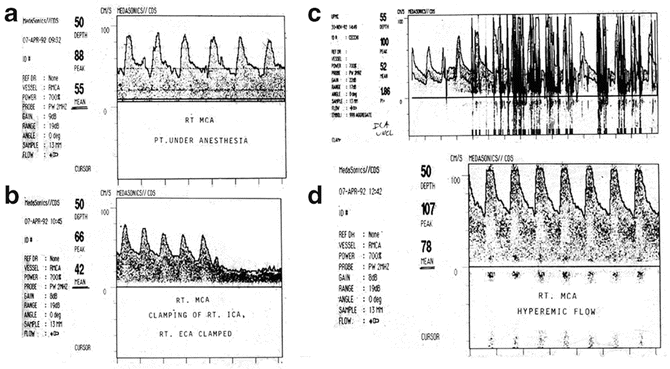
Fig. 46.2
Blood flow velocity measured by TCD during carotid endarterectomy. Preinduction (a). Internal and external carotid arteries clamped (b). Significant decrease in BFV occurs with loss of pulsatility (c). With unclamping significant emboli are observed in the middle cerebral artery. Postischemic hyperemia (d) is detected (From Kofke [165]; with permission)
Hyperemia
Hyperemia can be a major problem in some clinical conditions, as after AVM resection, after carotid endarterectomy (Fig. 46.2), with hepatic failure, and systemic hypertension. Given a baseline value or calibration of a TCD value with a CBF determination, TCD can be used to ascertain the presence of a cerebral hyperfusion syndrome. This has been reported after carotid endarterectomy by Steiger et al. [134, 135] and Lindegaard et al. [135]. Such syndromes risk the occurrence of so-called normal perfusion pressure breakthrough, wherein cerebral edema and/or hemorrhage can occur, despite the presence of normal blood pressure. After carotid endarterectomy, cerebral hyperemia is thought to predispose to postoperative cerebral hemorrhage, presumably as a consequence of impaired cerebral autoregulation [136]. Thus, TCD might be useful to judge the cerebral risk and titrate the need for aggressive prevention and/or treatment of systemic hypertension.
N ear-Infrared Spectroscopy Based Monitors of CBF
Recent reports indicate that noninvasive quantitative assessment of rCBF using near-infrared spectroscopy (NIRS ) should soon be available to clinicians for use in the operating room and ICU.
Kim et al. [137] recently described a NIRS-based system incorporating real-time assessment of diffuse correlation spectroscopy (DCS ) , a complex measure of erythrocyte motion, to derive a noninvasive, continuous quantitative index of rCBF . They validated it in animal studies with microspheres [138] and in preliminary studies of patients undergoing sequential XeCT CBF studies under various physiologic conditions associated with changes in CBF [137]. Blood flow under the patches measured by DCS correlated well with rCBFs assessed by the XeCT method. This group has, moreover, in animal [139] and human [140] studies, demonstrated the feasibility of using their technology to concurrently continuously measure CMRO2.
Concurrent with the earlier developments based on diffuse correlation spectroscopy, a group in Israel (Ornim, Inc.) is developing another system using NIRS plus ultrasound to derive a noninvasive continuous measure of rCBF and brain oxygen saturation. Proprietary data made available to the author suggest a correlation between the Ornim CerOx unit and thermodilution CBFs in humans undergoing hyperventilation. More peer-reviewed information will be needed and if supportive, this may indicate availability of another noninvasive quantitative regional CBF monitor.
These two lines of investigation suggest a realistic expectation that continuous CBF monitoring will soon be clinically available. Moreover, coupling this with continuous regional brain O2 saturation (RSO2) and oxygen extraction fraction (OEF ) information should permit concomitant CMRO2 monitoring, which will provide bedside information on whether the rCBF is properly coupled to metabolism. Finally, these monitors should also eventually provide continuous regional information on the presence of intact autoregulation. This will be an improvement on the below-described newly developed global monitors of autoregulation (for more information, see Chap. 12, “Near-Infrared Spectroscopy”) .
A rterial Spin Labeling
Defined as perfusion per unit of tissue, CBF is optimally measured with a diffusible tracer that can exchange between the blood and the brain. The currently established CBF imaging modalities include single-photon emission computed tomography (SPECT ) , positron emission tomography (PET ) , and XeCT CBF , all of which use radioactive diffusible tracers. Because none of these techniques can be applied routinely due to logistic or financial constraints, newly developed technologies, such as CT perfusion (CTP ) and arterial spin labeling (ASL) MRI, are becoming alternative standard imaging modalities for CBF evaluation and monitoring.
Applying a magnetic label as the diffusible tracer to blood water molecules, produced by saturating or inverting the longitudinal component of the MRI signal, ASL MRI provides quantitative CBF data and can be performed routinely and repeatedly without contrast administration or ionizing radiation. As labeling and imaging strategies evolve to improve the intrinsic limitations on signal-to-noise ratio per unit time and reduce several potential systemic measurement errors, ASL technique will likely become a standard MR sequence for CBF evaluation and monitoring [141].
Computed Tomography Perfusion
Computed tomography perfusion uses a nondiffusible contrast agent that is administered as a rapid intravenous bolus. Through sequential imaging to simultaneously record changes in the contrast agent concentration as a function of time, CTP generates data sets that principally include CBV, mean transit time (MTT ) , CBF, and time to peak (TTP ) [142, 143]. CTP can be rapidly obtained with simultaneous evaluation of cerebral arteries through CT angiography and does not require any particularly costly equipment, making it the imaging modality of choice for acute stroke evaluation. The extent of vertical brain coverage depends primarily on scanner detector configuration and the currently available 320 detector row scanners offer 16 cm slab of parenchyma. Because of the mathematical modeling employed and its use of a nondiffusible contrast agent, CTP -CBF data are currently regarded as qualitative rather than quantitative; however, relative perfusion values generated from contralateral reference parenchyma have demonstrated practical clinical utility, particularly in acute stroke imaging [144]. Active CTP research efforts are ongoing to reduce the innate radiation exposure and enhance its quantitative capacity [144, 145].
Intraoperative Cerebral Blood Flow Monitoring
Conventional methods for intraoperative assessment of blood vessel patency include digital subtraction angiography (DSA) and Doppler ultrasound and flowmetry . Although established as the gold standard for intraoperative vascular imaging, DSA requires substantial resources, additional operative time, and staff with a specific set of expertise to perform the procedure. Doppler ultrasound and flowmetry do not have high accuracy as an assessment tool for vessel lumen compromise [146]. Established noninvasive CBF imaging modalities such as PET are currently impractical for intraoperative uses due to logistical and financial constraints.
Presently, there is not yet a well-validated noninvasive intraoperative technology to not only visualize but also quantitatively measure cortical microcirculatory perfusion in sufficiently high temporospatial resolution. Using various intrinsic and extrinsic contrast agents, optical imaging modalities have emerged as the most promising intraoperative CBF monitoring techniques. Among such developing technologies, indocyanine green videoangiography (ICG-VA ) represents the most widely practiced intraoperative blood flow imaging tool.
Indocyanine Green Videoangiography
Introduced for neurovascular procedures more than 30 years ago [147, 148], fluorescence angiography has evolved to become a relatively mature and routinely applicable tool for simple, reliable, fast, and noninvasive intraoperative evaluation of blood flow. Indocyanine green (ICG) is a dye that gets injected into the bloodstream, subsequently excited with an infrared light source, and ultimately imaged with a camera that detects the fluorescence intensity. Because most modern surgical microscope systems have integrated ICG measurement capacities, this technology requires no additional intraoperative equipment but the actual ICG fluorescent dye for intravenous injection. It is commonly used during brain aneurysm clipping, intracranial–extracranial bypass, carotid endarterectomy, and resection of arteriovenous malformation and dural arteriovenous fistula [149].
ICG-VA uses fluorescence intensity as the principal contrast mechanism for imaging blood flow; therefore, this technology provides primarily qualitative information about blood flow, i.e., the presence or absence of blood flow in a vessel, and lacks quantitative measurement. FLOW 800 (Carl Zeiss, Oberkochen, Germany) was recently introduced as a microscope-integrated software tool that analyzes fluorescence intensity in a more detailed fashion [150, 151]. This software visually presents additional information on blood flow dynamics as a color-map and ICG intensity–time curve to identify direction of blood flow and relative timing of entry of ICG into exposed regions of brain. Although other optical imaging technologies such as laser speckle contrast imaging (LSCI ) provide more quantitative information about blood flow, they are not yet practical and easily available for intraoperative uses [152, 153].
The current ICG-VA technology displays fluorescence intensity as a white signal on a black background that makes the operative field not visible on the monitor. This represents another significant limitation of the technology. New techniques such as fluorescence angiography with augmented microscopy enhancement (FAAME ) are being developed to overlay the ICG signal onto the operative field so that an integrated presentation of real-time imaging of blood flow dynamics in an anatomically realistic background can be directly visible through the microscope oculars [154].
Monitors of Autoregulation
When cerebral autoregulation is intact, small changes in blood pressure will typically produce no changes in CBF, blood flow velocity, ICP , or brain oxygenation. These properties have been put to advantage in creating a number of approaches to continuously monitor the state of cerebral autoregulation by continuously calculating and monitoring the degree of mathematical correlation between ABP and these brain parameters. Dynamic time domain analysis of cerebrovascular autoregulation using TCD, ICP, PbO2, or NIRS-based regional oxygen saturation is a current topic of investigation with promising reports of potential efficacious and valid bedside use [35, 77, 78, 155, 156].
TCD-Based Autoregulation (Mx)
Recent advances in transcranial Doppler ultrasonograpy have allowed insights into dynamic, nearly instantaneous, assessment of cerebral autoregulation in critically ill patients. This approach determines the correlation coefficient between blood flow velocity (middle cerebral artery) and arterial blood pressure in real time at the bedside to make inferences regarding autoregulation. A high correlation of blood flow velocity with blood pressure suggests poor autoregulation, whereas no correlation is normal. Czosnyka et al. [34] observed in TBI patients a U-shaped curvilinear relationship in flow velocity versus ABP , with worse autoregulation (i.e., high correlation) with ABP less than 75 mmHg and ABP greater than 125 mmHg (Fig. 46.3). In another context, Joshi et al. [77], using TCD-based autoregulation assessment (Mx) in cardiac surgery patients reported an association of disturbed Mx with postoperative stroke [78, 79].
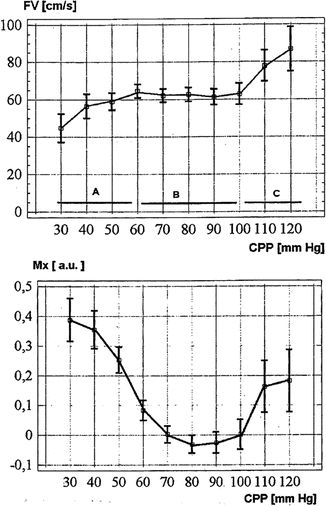
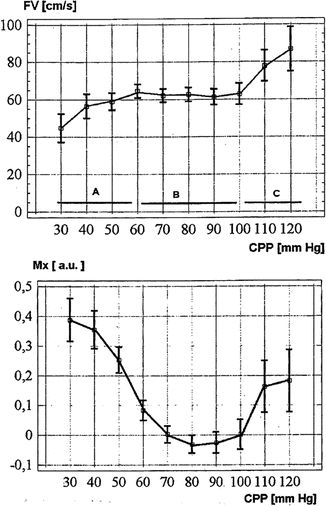
Fig. 46.3
Graphs demonstrating results of empirical regression of mean flow velocity by TCD (upper) and the Mx (a correlation coefficient) (lower) as they relate to CPP. Three distinctive zones of CPP—Zone A lying below the lower limit, Zone B lying within the range, and Zone C lying above the upper limit of autoregulation are marked. Vertical lines represent standard errors within CPP bins. a.u. arbitrary units, s second (From Czosnyka et al. [34]; with permission)
ICP -Based Autoregulation (Prx)
Intracranial pressure ordinarily does not vary with changes in blood pressure within the normal autoregulatory range. However, with injury-induced cerebral dysautoregulation, ICP will vary with ABP such that information on the state of cerebral autoregulation can be inferred. The ICP pressure-reactivity index (PRx) is a described quantitation of the aforementioned description of abnormal dynamic correlation of ICP changes with ABP changes and is another means to dynamically evaluate autoregulation [35–37, 157], with reports indicating that Prx correlates well with other autoregulation indices [36, 37, 158, 159]. Steiner et al. [35] reported on the use of Prx monitoring in TBI patients to determine the optimal CPP. Patients with better autoregulation in this optimal range as defined by Prx had better outcomes. Moreover, patients with dysautoregulation related to higher ABP with corresponding ICP elevation also had worse outcomes, suggesting that autoregulation monitoring, to ensure adherence to an individual’s optimal CPP, may be an outcome-altering ICU measure. Zweifel et al. [36] report congruent observations. Notably, Prx, as with TCD-based autoregulation studies, also appears to undergo a U-shaped curvilinear relationship with variations in CPP, with it being abnormally high (i.e., ICP varies with ABP ) at low (ischemic) and high (hyperemic) CPP in TBI patients. Moreover, further complementing this are observations of abnormally high OEF and low OEF at these respective ABP extremes. This is underscored by reports of a significant ischemic burden in TBI patients [160–163], suggesting a delicate balance between hypotension-associated hypoperfusion and hypertension-associated edema/ICP exacerbation, both of which will worsen regional ischemia. Taken together, these autoregulation studies introduce the notion that there is an individualized ABP optimum in TBI patients [36] that should be a therapeutic goal .
COx
Autoregulation monitoring is also being reported using brain near infrared spectroscopy (COx), suggesting that noninvasive autoregulation assessment will be a feasible bedside modality that can be used to deliver the optimal CPP. Joshi et al. [77] reported, in cardiac surgery patients, an association of disturbed Mx with postoperative stroke with good agreement between Mx and Cox, thus demonstrating feasibility of COx assessed with NIRS [78, 79]. Finally, brain PbO2 has been reported to also be amenable to use as a regional autoregulation monitor (ORx) [80].
All of these autoregulation indices appear to be feasible to use to monitor and individualize CBF in the operating room. Initial studies in the cardiac surgery [77, 79], and sitting orthopedics [164] contexts support a potential role for brain autoregulation monitoring in neurosurgery and other operative settings, which may help guide blood pressure management.
Conclusion
In summary, many different technologies are now available to provide monitoring information regarding cerebral blood flow in critically ill patients during and after surgery. The available techniques provide a range of options in terms of quantitation, anatomic resolution, portability, and speed of information availability. Unfortunately, no perfect monitor is yet available that provides all of the needed attributes and makes the data easily available at the bedside in the ICU or OR. Nonetheless, the available methods have provided for significant advances in our approach to thinking about and managing cerebral blood flow in the critically ill.
References
2.
Kuschinsky M. Coupling of blood flow and metabolism in the brain. J Basic Clin Physiol Pharmacol. 1990;1:191–201.PubMed
3.
Meyer J, Shimazu K, Okamoto S, et al. Effects of alpha adrenergic blockade on autoregulation and chemical vasomotor control of CBF in stroke. Stroke. 1973;4:187.PubMed
4.
Owman C, Edvinsson L, Hardebo J. Pharmacological in vitro analysis of amine-mediated vasomotor functions in the intracranial and extracranial vascular beds. Blood Vessels. 1978;15:128.PubMed
5.
Suzuki N, Hardebo J. The cerebrovascular parasympathetic innervation. Cerebrovasc Brain Metab Reb. 1993;5(1):33.
6.
Meglio M, Cioni B, Visocchi M, et al. Spinal cord stimulation and cerebral haemodynamics. Acta Neurochir (Wien). 1991;111(1–2):43.
7.
Garnett E, Nahmias C, Scheffel A, Firnau G, Upton A. Regional CBF in man manipulated by direct vagal stimulation. Pacing Clin Electrophysiol. 1992;15:1579.PubMed
8.
Sato A, Sato Y. Regulation of regional CBF by cholinergic fibers originating in the basal forebrain. Neurosci Res. 1992;14:242.PubMed
9.
Faraci F, Brian Jr J. Nitric oxide and the cerebral circulation. Stroke. 1994;25:692.PubMed
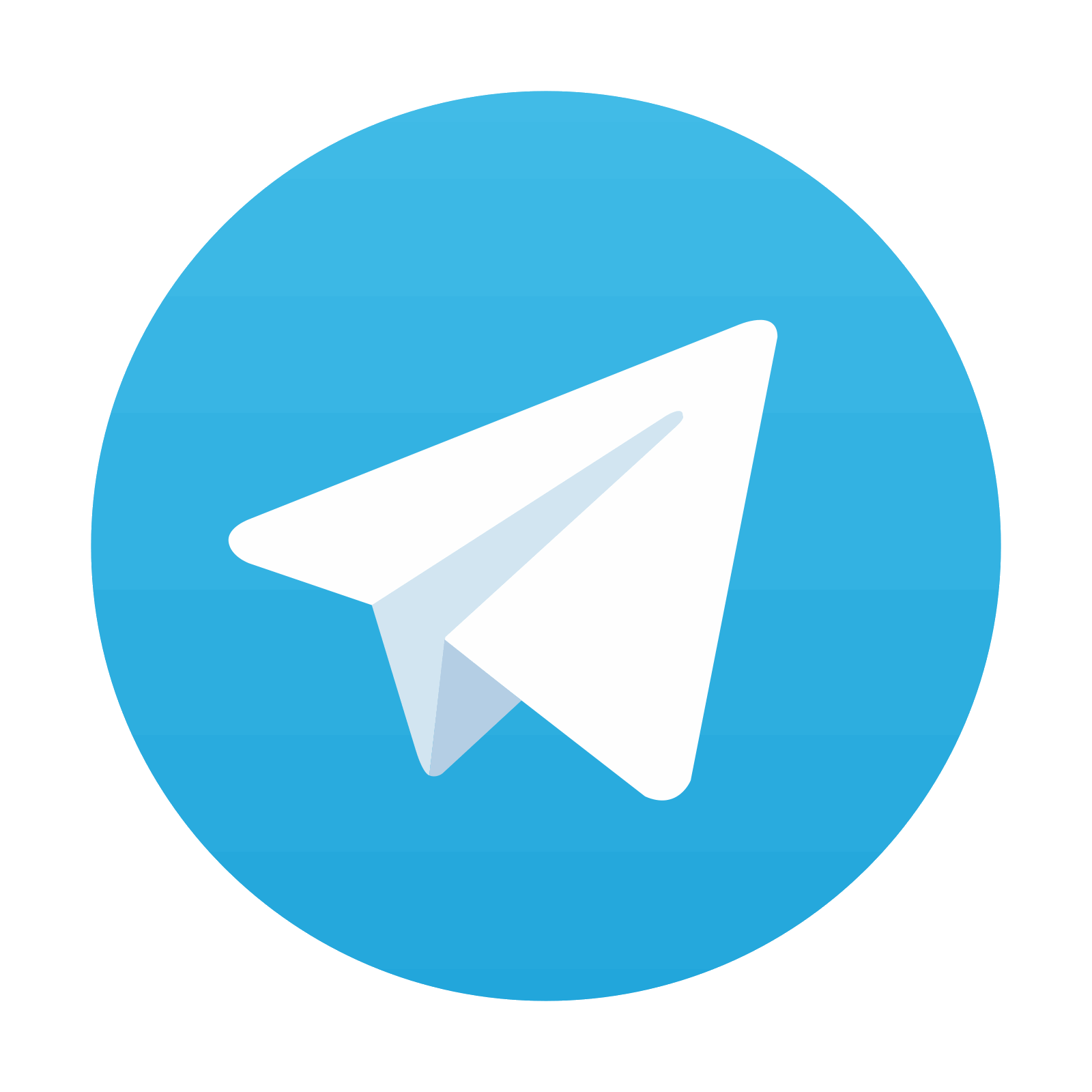
Stay updated, free articles. Join our Telegram channel
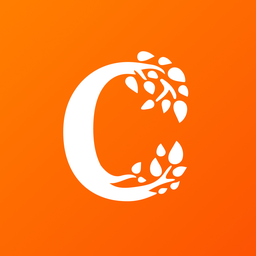
Full access? Get Clinical Tree
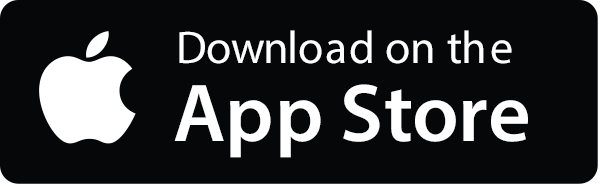
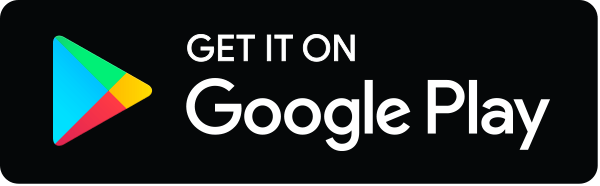