![]() FIGURE 5.1 Gate control theory as originally schematically described by Melzack and Wall2 where large-diameter (L) and small-diameter (S) primary afferent fibers project to substantia gelatinosa (SG) and second-order transmission (T) neurons in the spinal dorsal horn. The inhibitory effect of SG neuronal activity is increased by L and decreased by S fiber activity. T neurons transmit information to the brain and other action sites. Activation of peripheral or central projections of L fibers using transcutaneous nerve stimulation, peripheral nerve stimulators, or dorsal column stimulators would all be expected to produce inhibition of S fiber input to the T cells. |
lability can occur. The precise neurophysiologic circuits associated with this descending inhibition is of significant debate, but known inhibitory neurotransmitters such as norepinephrine (NE) and serotonin (5-hydroxy-tryptophan; 5-HT) are synthesized in the brainstem and transported to the spinal cord from multiple supraspinal sites. This role for supraspinal structures in providing descending influences on spinal reflexes has long been recognized. In 1915, Sherrington and Sowton12 demonstrated enhanced flexion reflexes following spinal transection. Later in 1926, Fulton13 suggested that this effect reflected removal of tonic descending inhibitory modulation of spinal interneurons mediating those reflexes. Descending control of flexion reflexes was extensively studied in ensuing years,14 but these studies did not target the issue of how the brain might specifically modulate incoming nociceptive signals from peripheral tissue.
midbrain reticular formation, locus coeruleus/A6 cell group (LC/A6), the A5 cell group, the lateral reticular nucleus (LRN) and nearby A1 and C1 cell groups, the parabrachial nucleus/A7 cell group (PBN/A7) and the nucleus tractus solitarius (NTS). A limited amount of information is also available on cortical and limbic systems such as the anterior cingulate cortex (ACC), the amygdala, and hypothalamus that contribute to descending modulation. It is the investigation of these structures that has led to our current understanding of how descending pain modulatory systems affect pain perception. This topic has been well reviewed.1,23,24,25,26,27,28,29 Table 5.1 summarizes results related to many of these areas separately along with the neurotransmitters associated with their putative inhibitory versus facilitatory effects on nociceptive transmission. A summary of the most important components is described in Figure 5.5.
TABLE 5.1 Central Nervous System (CNS) Sites Modulating Nociceptive Transmission (NT) | ||||||||||||||||||||||||||||||||||||||||||||||||||||||||||||||||||||||||||||||||||||||||||||||||||||||||||||||||||||||
---|---|---|---|---|---|---|---|---|---|---|---|---|---|---|---|---|---|---|---|---|---|---|---|---|---|---|---|---|---|---|---|---|---|---|---|---|---|---|---|---|---|---|---|---|---|---|---|---|---|---|---|---|---|---|---|---|---|---|---|---|---|---|---|---|---|---|---|---|---|---|---|---|---|---|---|---|---|---|---|---|---|---|---|---|---|---|---|---|---|---|---|---|---|---|---|---|---|---|---|---|---|---|---|---|---|---|---|---|---|---|---|---|---|---|---|---|---|---|
|
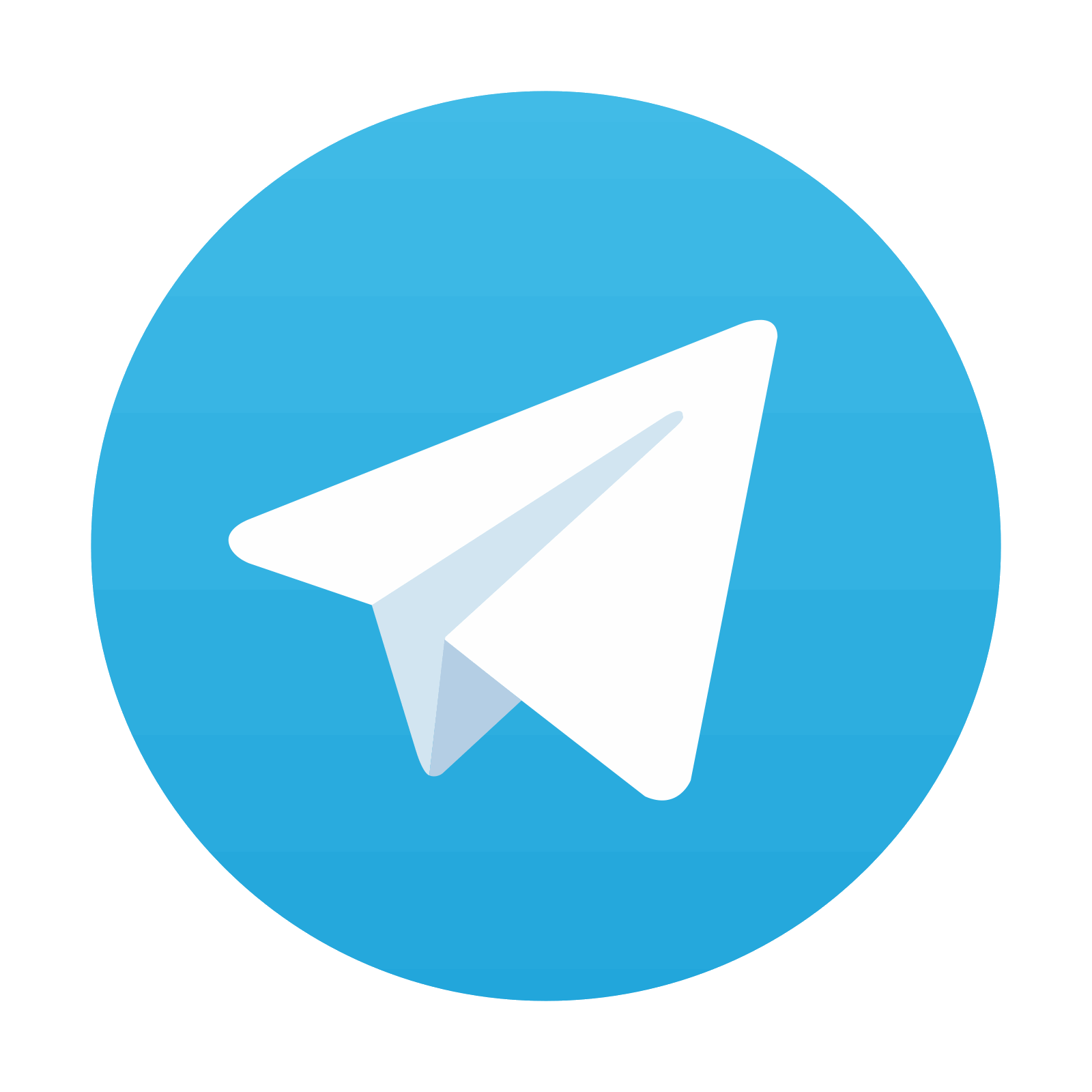
Stay updated, free articles. Join our Telegram channel
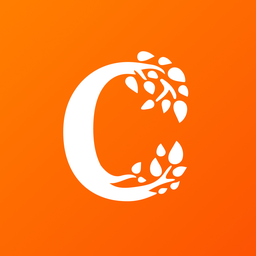
Full access? Get Clinical Tree
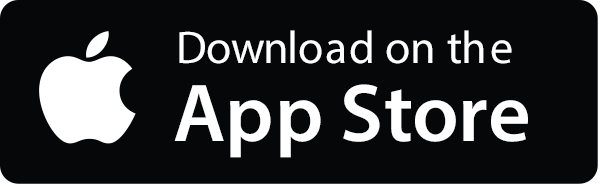
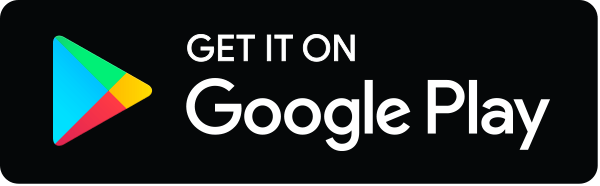
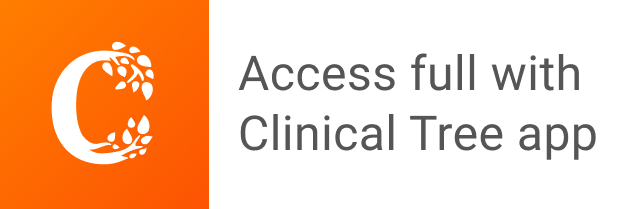