Minimally Invasive Cardiology
Andrew J. Goodwin
Ednan K. Bajwa
Atul Malhotra
The assessment of cardiac output has historically been vital to the management of critically ill or hemodynamically unstable patients. The underlying nature of shock in a hypotensive patient may not be obvious and is often multifactorial. In these circumstances, it is crucial to characterize what type of shock (i.e., distributive, cardiogenic, hypovolemic) is playing a role in a patient’s presentation as well as how he or she will respond to interventions, such as volume loading. Determination of cardiac output is thought to be a critical component of this process and has been the center of research for decades.
The physical examination has proven to be a largely unreliable means of assessing hemodynamics in systolic heart failure [1] and in critically ill patients without recent myocardial infarction [2]. As such, more dependable measurements may be required to optimally treat such patients. Since its introduction [3], the pulmonary artery catheter (PAC) has been useful in obtaining measurements of cardiac output and has been used both diagnostically as well as to gauge response to treatment. For many years, the thermodilution PAC was considered to be the “gold standard” of intensive care unit (ICU) hemodynamic measurement. This philosophy has been called into question during the last several years in light of mounting evidence that clinicians may be using the PAC ineffectively [4] and that morbidity and mortality in a variety of clinical situations are not improved with its use [5,6], but instead may be worsened [7,8].
In light of these studies, many clinicians have begun to question the importance and the credibility of the PAC. Some postulate that the lack of improvement on morbidity and mortality stems from the deleterious complications that are inherently associated with an invasive procedure. Others have shown that even when the balance of oxygen supply and demand in critically ill patients is known and is optimized, even to supranormal levels, there is no improvement in outcomes [9]. This gives rise to the notion that once tissue hypoperfusion results in organ dysfunction, a cycle of inflammation ensues that leads to irreversible organ damage if not corrected early. This concept has been described as “cytopathic hypoxia,” wherein hypoperfusion leads to the disruption of the intracellular utilization of oxygen to the point that delivery of normal or supranormal amounts of oxygen to a cell will not restore its function [10,11]. Some data have emerged that suggest that correction of hypoperfusion and inadequate oxygen delivery early in the course of sepsis yields beneficial mortality outcomes [12,13]. Interestingly, these studies did not use PACs, but instead used central venous oxygen saturation as a surrogate for cardiac output and volume responsiveness.
While we await more data to clarify the usefulness of directly monitoring cardiac output in critically ill patients and the timing at which it is best to use this monitoring, many are focusing on alternative and less-invasive methods of determining cardiac function. These methods can be divided into two broad categories: Measurements of cardiac output and measurements of indices of oxygen delivery and/or tissue perfusion as surrogates for cardiac output. The goal of this research has been to develop minimally invasive yet reliable and accurate techniques that can be easily applied to critically ill patients. In some cases, these studies have focused on adapting monitoring technology that is already routinely used in this patient population.
In this chapter, we focus on several emerging technologies being used to determine cardiac output and tissue perfusion in the critically ill patient. The bulk of the discussion will involve the methods of Doppler echocardiography, pulse contour analysis (PCA), partial carbon dioxide rebreathing, and gastric tonometry as these represent the modalities best studied to date. Consideration will also be given to new and developing methods such as thoracic bioimpedance, sublingual capnometry, and biomarkers. We will conclude with a summary of practice recommendations and future directions.
Cardiac Output
Cardiac output is the amount of blood flow through the cardiovascular system during a period of time. Traditionally, it is reported in liters per minute and can be normalized for a person’s body surface area to provide the cardiac index. In the healthy subject, cardiac output is directly related to that subject’s metabolic rate and oxygen consumption ([V with dot above]o2). The therapy for a hypotensive patient with diminished cardiac output (cardiogenic shock) is fundamentally different from the therapy for a patient with diminished systemic vascular resistance (distributive shock). Therefore, an accurate knowledge of these variables is vital to the effective treatment of the hypotensive, critically ill patient. As the systemic vascular resistance can be challenging to measure directly, it is calculated from the ratio of pressure gradient (mean arterial pressure minus central venous pressure) and flow rate (cardiac output). This formula assumes an ohmic resistor (i.e., one with a linear pressure-flow relationship). Because a fall in systemic vascular resistance could represent a decrease in blood pressure or a rise in cardiac output, we favor the use of the primary measured variables in hemodynamic assessments. We would also caution against the use of the
systemic vascular resistance in isolation, without consideration for the factors leading to its fluctuations (e.g., changes in cardiac output).
systemic vascular resistance in isolation, without consideration for the factors leading to its fluctuations (e.g., changes in cardiac output).
Traditionally, a number of techniques have been used for the assessment of cardiac function. Jugular venous pulsations, the presence of an S3 gallop, and skin temperature have all been studied as means of using the physical examination to estimate cardiac output with mixed results [14,15 and 16]. The pulmonary artery occlusion pressure (PAOP) and central venous pressure (CVP) have also been used as surrogates for left and right ventricular function, respectively. The PAOP is commonly used to establish the diagnosis of left heart failure in the hypotensive patient and is often used to guide resuscitation. Magder and colleagues [17,18] demonstrated that the CVP could provide useful information about the volume status of critically ill patients. Because the majority of the blood volume is in the systemic veins, and the right ventricle is the major determinant of cardiac output, some would argue that the CVP should receive more attention as the focus of hemodynamic resuscitation protocols. Unfortunately, PAOP and CVP only represent the end-diastolic pressures of their respective chambers. These variables do not always accurately translate into systolic function and cardiac output. In addition, invasive assessment of PAOP [19,20] and clinical assessment of CVP [21] have been notoriously difficult to assess accurately and reliably.
During the last few decades, considerable research has been devoted to the accurate measurement of cardiac output by minimally invasive means. At present, there exist several modalities that are able to provide estimates of cardiac output on a continuous or near-continuous basis. Each is based on unique strategies and each has notable advantages and disadvantages. Although some have been established enough to warrant increasing use in clinical settings (esophageal Doppler [ED], PCA), the clinical usefulness of others is still being determined (partial carbon dioxide rebreathing, thoracic bioimpedance). Here, we describe each of these four modalities and summarize the evidence regarding their use.
Esophageal Doppler
To date, the ED has been one of the most rigorously studied noninvasive cardiac output measurement modalities. It was first described by Side and Gosling [22] in 1971 and was later refined by Singer et al. [23]. This technique uses a Doppler probe placed in the esophagus to measure blood flow in the descending thoracic aorta. The ED uses the Doppler shift principle, which implies that when a transmitted sound wave is impeded by a structure, the reflected sound wave will vary in a frequency-dependent manner with the structure’s characteristics. In the case of a fluid-filled tube, such as the aorta, the magnitude of Doppler shift will vary in direct proportion to the velocity of flow in the tube (Fig. 29-1). Thus, the reflected sound wave can be used to determine flow velocity in the descending aorta. Multiplying this flow velocity by the ejection time and the cross-sectional area of the aorta provides an estimate of the stroke volume (SV). As this measurement does not account for the component of total SV that travels to the coronary, carotid, and subclavian arteries, a correction factor must be applied to estimate the total SV. Cardiac output is then calculated by multiplying corrected SV by the heart rate. The original versions of the ED system only provided Doppler shift data; therefore, the cross-sectional area of the aorta was estimated from a nomogram based on a patient’s height, weight, and age. Subsequently, a newer model with both a Doppler and ultrasound probe has been introduced that can simultaneously provide estimates of both aortic flow velocity and cross-sectional area [24]. The descending aortic cross-sectional area measured by this model correlated very well with that measured by transesophageal echocardiography. In addition, the aortic blood flow measured with this model was also well correlated with cardiac output as measured by thermodilution [24].
Beyond providing an estimate of cardiac output, ED systems can provide information about the preload and the contractility of the heart. Singer et al. [23] and Singer and Bennett [25] analyzed the flow-velocity waveform derived from an ED system and discovered that the corrected flow time correlated with preload (Fig. 29-2). These studies further demonstrated that as preload was increased or decreased, the corrected flow time increased or decreased, respectively [23,25]. Wallmeyer and colleagues [26] described a correlation between the peak velocity measured by a Doppler and contractility measured by electromagnetic catheter measured flow. Singer et al. [27] further substantiated this finding by demonstrating that dobutamine infusions increased peak flow velocities measured by an ED system in a dose-dependent fashion. These observations suggest that an experienced operator may be able to extrapolate useful hemodynamic parameters, beyond the cardiac output, through careful interpretation of the generated data.
Clinical Utility
The clinical usefulness of the ED system is still being determined. The majority of recent studies that have compared this system to the gold standard of thermodilution have been performed in either intraoperative or postoperative settings and have revealed mixed results. One single-center study of 35 patients that compared ED measurements of cardiac output with simultaneous measurements of cardiac output by thermodilution during off-pump coronary artery bypass grafting showed very poor
correlation between the two techniques [28]. Other studies, including a metaanalysis of 11 trials, have shown that ED systems are better at following changes in cardiac output in response to fluid challenges than they are at measuring the absolute cardiac output [29,30 and 31]. The authors of the metaanalysis also made an important point when discussing the reliability of comparing ED systems with thermodilution. They argued that the poor reproducibility inherent to the use of the thermodilution technique will likely affect the limits of agreement between ED systems and thermodilution, irrespective of the performances of the ED systems [29]. Stated simply, because the measurements of cardiac output by thermodilution vary significantly even when drawn temporally close to each other, ED systems will not have close agreement to thermodilution systems even if their measurements are reliable. This concept was described by Bland and Altman [32] and has important implications when trying to interpret the degree of accuracy of absolute cardiac output measured by any system when compared to thermodilution.
correlation between the two techniques [28]. Other studies, including a metaanalysis of 11 trials, have shown that ED systems are better at following changes in cardiac output in response to fluid challenges than they are at measuring the absolute cardiac output [29,30 and 31]. The authors of the metaanalysis also made an important point when discussing the reliability of comparing ED systems with thermodilution. They argued that the poor reproducibility inherent to the use of the thermodilution technique will likely affect the limits of agreement between ED systems and thermodilution, irrespective of the performances of the ED systems [29]. Stated simply, because the measurements of cardiac output by thermodilution vary significantly even when drawn temporally close to each other, ED systems will not have close agreement to thermodilution systems even if their measurements are reliable. This concept was described by Bland and Altman [32] and has important implications when trying to interpret the degree of accuracy of absolute cardiac output measured by any system when compared to thermodilution.
![]() FIGURE 29-2. Esophageal Doppler flow-velocity waveform. [Adapted from Marik PE: Pulmonary artery catheterization and esophageal Doppler monitoring in the ICU. Chest 116:1085, 1999, with permission.] |
TABLE 29-1. Advantages and Disadvantages of the Esophageal Doppler System for Cardiac Output Monitoring | |
---|---|
|
Advantages and Disadvantages
While comparing ED systems to thermodilution, it is also important to consider the technical advantages and disadvantages (Table 29-1). One advantage of the ED system is that it is continuous. Unlike the traditional bolus thermodilution techniques, an ED system can continuously display cardiac output, which allows earlier recognition of hemodynamic deterioration or improvement in system responsiveness to a therapeutic intervention. Additionally, an ED probe can be placed in minutes and has not been associated with important iatrogenic complications [33,34]. Some in situ data suggest that, once inserted, an esophageal probe can be left in place safely for more than 2 weeks [35]. One study determined that the training required to become proficient in the use of ED consisted of no more than 12 patients [36]. Furthermore, as the esophagus is a nonsterile environment, it is logical to assume that the infectious risk of ED probe use is less than that of a PAC placed in normally sterile tissues.
There are also technical disadvantages to the ED system. One is the high up-front cost of the system itself as compared to the PAC apparatus. This cost may represent a very real limitation in the number of systems that a facility can purchase and maintain. This financial obstacle must be balanced with the likelihood that multiple patients would have need of this system simultaneously, which would necessitate multiple systems. Another disadvantage of this system is that it can be used only in the intubated patient. Although a large percentage of critically ill and/or surgical patients who would benefit from this system fit this criterion, the nonintubated patient would have to be excluded as a candidate. Additional concerns would include the likely need for repositioning or recalibrating the ICU patient. Although a surgical patient is often paralyzed and remains immobile, ICU patients are often repositioned frequently in order to prevent skin breakdown or to facilitate improved oxygenation. Such movements will increase the chance of probe position changes that will require frequent calibration and repositioning. Finally, Roeck et al. [31] suggested that there is significant interobserver variability when measuring changes in SV in response to fluid challenges with ED. Without a reproducible interpretation of data, the clinical usefulness of the ED system will be limited. We also believe that extensive clinical experience with this technique is important and would caution against the implementation of ED technique without adequate training.
Future Research
As the ED is used more widely, it will be critical to establish whether its use will actually improve patient care. To date, the majority of research on this technique has focused on validating its data and determining if it is a technically feasible modality of measuring cardiac output. Very little data exist at present about whether the use of this technique affects patient outcomes. One notable study that compared intraoperative ED use with “conventional” monitoring during femoral neck fracture repair found a faster recovery time and significantly shorter hospital stay in the ED group [37]. Although this study is encouraging, its results have not been reliably repeated as of yet. As previous trials have shown us, even the gold standard has not had a positive effect on patient care [7,8]. Future research on ED should begin to focus more on outcome data.
Pulse Contour Analysis
PCA is another modality for measuring cardiac output noninvasively that has been extensively studied. This method relies on the theory, first described by Frank [38] in the early part
of the 20th century, that SV and cardiac output can be derived from the characteristics of an aortic pressure waveform. Wesseling and colleagues [39] eventually published in 1983 an algorithm to mathematically link SV and the pressure waveform. This original version calculated SV continuously by dividing the area under the curve of the aortic pressure waveform by the aortic impedance. Because aortic impedance varies between patients, it had to be measured using another modality to initially calibrate the PCA system. The calibration method usually employed was arterial thermodilution. Aortic impedance, however, is not a static property. It is based on the complex interaction of the resistive and compliant elements of each vascular bed, which are often dynamic, especially in hemodynamically unstable patients. Since the first PCA algorithm was introduced, several unique algorithms have been created in an attempt to accurately model the properties of the human vascular system for use in PCA systems.
of the 20th century, that SV and cardiac output can be derived from the characteristics of an aortic pressure waveform. Wesseling and colleagues [39] eventually published in 1983 an algorithm to mathematically link SV and the pressure waveform. This original version calculated SV continuously by dividing the area under the curve of the aortic pressure waveform by the aortic impedance. Because aortic impedance varies between patients, it had to be measured using another modality to initially calibrate the PCA system. The calibration method usually employed was arterial thermodilution. Aortic impedance, however, is not a static property. It is based on the complex interaction of the resistive and compliant elements of each vascular bed, which are often dynamic, especially in hemodynamically unstable patients. Since the first PCA algorithm was introduced, several unique algorithms have been created in an attempt to accurately model the properties of the human vascular system for use in PCA systems.
PCA involves the use of an arterially placed catheter with a pressure transducer, which can measure pressure tracings on a beat-to-beat basis. Such catheters are now routinely used in operating rooms and ICUs as they provide a continuous measurement of blood pressure that is believed to be superior to intermittent noninvasive measurements in hemodynamically unstable patients. These catheters are interfaced with a PCA system, which uses its unique algorithm as well as the initial aortic impedance calibration data from a thermodilution measurement of cardiac output to provide a continuously displayed measurement of cardiac output. Obviously, the reliability of a PCA system depends on the accuracy of the algorithm that it employs. Because each algorithm is unique in the weight that it ascribes to each element of vascular conductivity, it is impossible to ensure that a system will be able to reproduce the results of another system under similar conditions [40]. Keeping this in mind, one cannot conclude that all systems are equally reliable.
One particular PCA system has received considerable attention in the literature. Numerous studies have demonstrated good correlation between this system and pulmonary thermodilution in both critically ill and surgical patients [41, 42, 43, 44 and 45]. Notably, this system did not require recalibration during these study periods, which were performed under static ventricular loading conditions. The system involves the placement of a femoral arterial catheter that is passed into the abdominal aorta. In addition to a pressure transducer, the catheter also contains a thermistor for arterial thermodilution. The system is calibrated by injecting cold saline via a central venous catheter at the right atrium in a manner similar to pulmonary arterial thermodilution. Instead of using a thermistor in the pulmonary artery, however, the thermistor on the arterial catheter allows calculation of cardiac output. This initial value of cardiac output is then used to calibrate the PCA system that is attached to the arterial catheter. Because the arterial catheter is used for calibration, a PAC is not necessary. When compared to pulmonary artery thermodilution, the arterial thermodilution method was found to be accurate, implying that it is an acceptable method for calibration of a PCA system [41,42 and 43].
Clinical Utility
As mentioned previously, the initial trials studying this system used data from static ventricular loading conditions. Both the critically ill and the surgical patient, however, often experience rapid changes in ventricular preload. The validity of this system with changes in preload was addressed in a subsequent study, which used an updated version of the previous algorithm. Felbinger and colleagues [46] showed that changes in cardiac output in response to preload could be accurately measured in a cardiac surgical ICU population when compared to pulmonary thermodilution.
Although being able to monitor cardiac output during volume loading is important, being able to determine when a patient would benefit from increased preload has major clinical benefit. Pulse pressures commonly vary throughout the respiratory cycle. Pulse pressure variation (PPV) is defined as the result of the minimum pulse pressure subtracted from the maximum pulse pressure divided by the mean of these two pressures.
|
It has been observed that the magnitude of the pulse pressure variation in a patient can predict preload responsiveness [47,48 and 49]. Analogous to pulse pressure variation, an additional piece of data that PCA systems can provide is the stroke volume variation (SVV). The SVV represents the change in percentage of SV over a preceding time period as a result of changes in SV due to ventilation. Thus far, the ability to use SVV to determine preload responsiveness has yielded mixed results. Reuter et al. [50] found that SVV reliably decreased as cardiac index increased in response to preloading with colloids in ventilated postoperative cardiac surgical patients. This finding supports the argument that the magnitude of SVV may be used to predict preload responsiveness. It is important to note that the tidal volumes used in this study were supraphysiologic (15 mL/kg), which results in a larger SVV and a resultant increase in the accuracy of this approach. Subsequently, another study used a smaller tidal volume (10 mL/kg) in a similar patient population and could not demonstrate a reliable relationship between SVV and an increase in cardiac index in response to preloading [51]. This finding suggests that when using lower tidal volume ventilation strategies, which are optimal for acute respiratory distress syndrome (ARDS), PCA-derived SVV should not be used to estimate preload responsiveness.
Advantages and Disadvantages
Overall, the PCA system offers several advantages over the traditional gold standard of pulmonary artery thermodilution (Table 29-2). It requires the use of an arterial catheter as well as a central venous catheter, which are both commonly already in place in critically ill and surgical patients for monitoring and therapeutic purposes. Because these systems can be reliably calibrated by the arterial thermodilution method, the potential risk of a PAC can be avoided. The PCA system also provides a continuous measurement of cardiac output as opposed to the intermittent nature of traditional thermodilution systems.
As with any system, there are disadvantages to the PCA system as well. The ability to use this system to determine preload responsiveness is questionable in patients who are being managed with common ventilatory strategies. In addition, some data suggest that in patients who have marked changes in blood pressure, the algorithm is not able to model adequately the changes in vascular resistance and compliance and, therefore, the accuracy of the measured cardiac output declines [52]. Furthermore, a similar breakdown in the accuracy of measured cardiac output has
been suggested during the administration of vasoconstrictors [53], which are common in the critically ill patient.
been suggested during the administration of vasoconstrictors [53], which are common in the critically ill patient.
TABLE 29-2. Advantages and Disadvantages of the Pulse Contour Analysis Method for Cardiac Output Monitoring | |
---|---|
|
Future Research
The clinical utility of the PCA system is still being determined. Future studies that may help in defining the system’s clinical role could focus on several points. First, a better understanding of how changes in blood pressure and vasoconstrictor use affect the accuracy of a particular system’s algorithm will help to determine a schedule for when a system needs to be recalibrated in order to maintain its accuracy. Additionally, an analysis of how SVV predicts preload responsiveness at tidal volumes that are commonly used in the ARDS patient population will provide more applicable information than what is currently available. Finally, a paucity of data regarding how PCA systems affect patient outcomes exists at present. Comparisons between the outcomes seen with this system and pulmonary artery thermodilution may provide convincing evidence about the real usefulness of PCA.
Partial Carbon Dioxide Rebreathing Method
The Fick equation for calculating cardiac output has been known for more than 100 years. Its underlying principle states that for a gas (X) whose uptake in the lung is transferred completely to the blood, the ratio of that gas’s consumption (VX) to the difference between the arterial (CaX) and venous (CvX) contents of the gas will equal the cardiac output. In its original form, Fick used the example of oxygen (o2) and described the following equation:

For this equation to be accurate, several conditions must exist. The first is that blood flow through the pulmonary capillaries must be constant. In order for this to occur, the right and left ventricular outputs must be equal and there must be no respiratory variation of pulmonary capillary flow. Another condition critical to this method’s accuracy is an absence of shunts. As this method depends on using gas exchange to calculate cardiac output, any blood that does not participate in gas exchange will result in underestimation of cardiac output. Furthermore, oxygen uptake by the lung itself must be minimal to maintain the integrity of this equation.
Although possible, the accurate measurement of [V with dot above]o2 is clinically challenging, especially in patients who require high Fio2[54]. This prompted some investigators to focus on using carbon dioxide production ([V with dot above]co2) in place of [V with dot above]o2 [55,56 and 57]. As [V with dot above]o2 is equal to [V with dot above]co2 divided by the respiratory quotient, they determined that cardiac output could be calculated by [V with dot above]co2 divided by the arteriovenous difference between O2 concentrations as well as the respiratory quotient (R). In order to measure O2 concentrations continuously, arterial and venous oximeters were used to measure oxygen saturation (So2) and concentration was determined based on measured hemoglobin (Hgb) levels. This technique, therefore, relied upon the assumption that both R and Hgb levels remained constant during the measurement period.
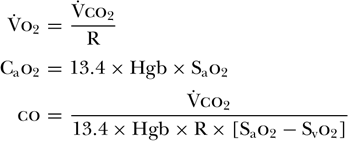
Using this method, one study found good correlation with cardiac output determined by thermodilution [57]. The drawback to this approach, however, is the need for an invasive central venous catheter to accurately measure venous oxygen saturations as well as to initially calibrate the system and determine R. Subsequently, the partial carbon dioxide rebreathing method was introduced in an attempt to avoid the need for such catheters.
The partial co2 rebreathing method is based upon the Fick equation for co2 [58]:
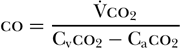
When using this method, a disposable rebreathing loop is placed between the endotracheal tube and the ventilator, resulting in the rebreathing of carbon dioxide. A carbon dioxide sensor, an airflow sensor, and an arterial noninvasive pulse oximeter are then used to gather data before and after a period of co2 rebreathing. The co2 sensor and airflow monitor allow for the calculation of [V with dot above]co2 both before and during the rebreathing period. Because cardiac output does not change from baseline during rebreathing conditions [59], one can generate the following equation [58]:

Gedeon et al. [60] determined that subtracting the rebreathing ratio from the baseline ratio yields the following equation [58]:

As co2 diffuses rapidly into the blood, one can further assume that the mixed venous co2 concentration (Cvco2) remains unchanged between baseline and rebreathing conditions; that is, Cvco2 baseline = Cvco2 rebreathing. This allows for further simplification of the equation to the following [58]:
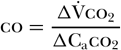
Caco2 can be estimated from end-tidal carbon dioxide (etco2) and the carbon dioxide dissociation curve. Therefore, δCaco2 can be substituted for by δetco2 multiplied by the slope (S) of
the dissociation curve [58]:
the dissociation curve [58]:
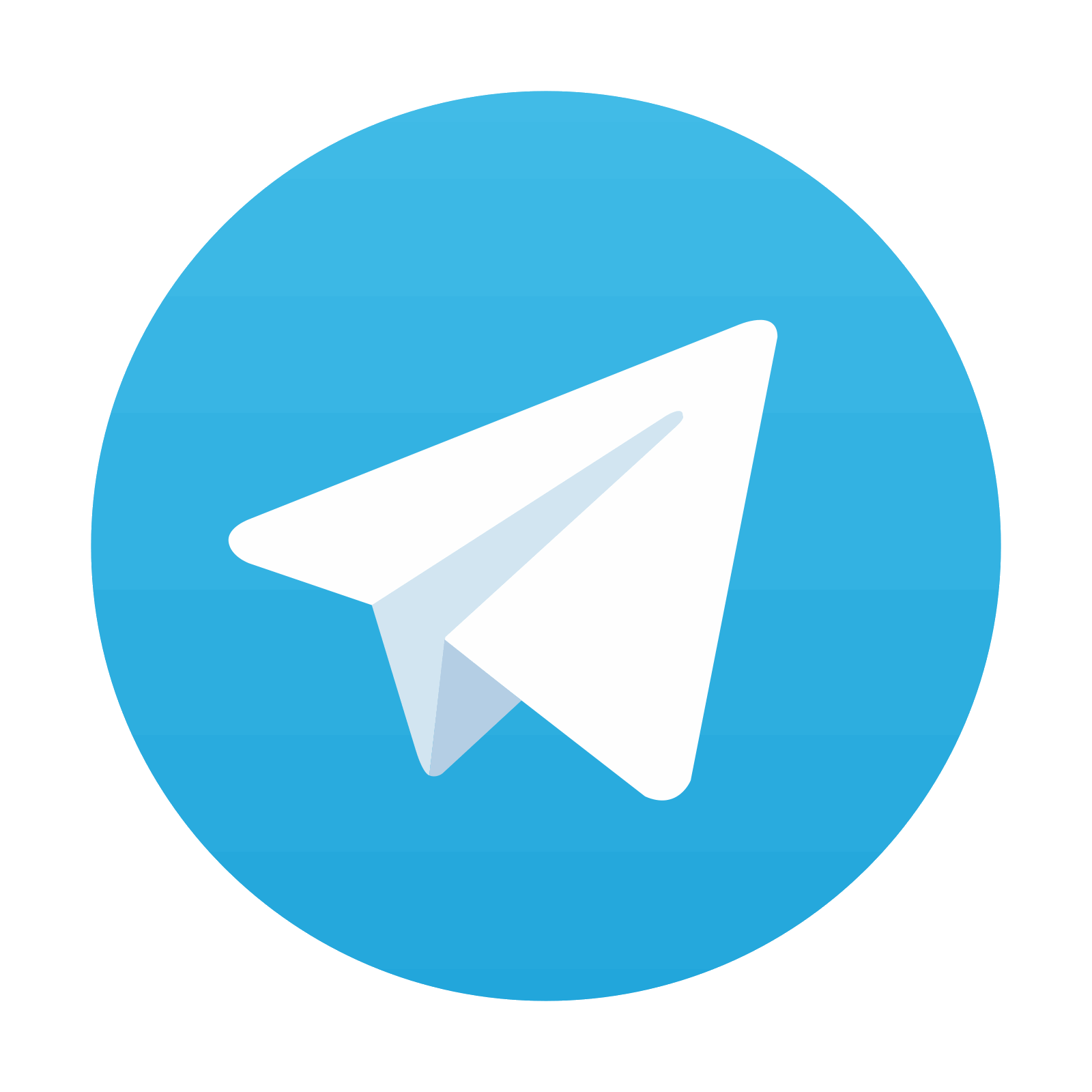
Stay updated, free articles. Join our Telegram channel
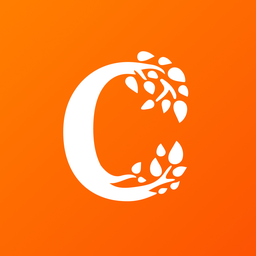
Full access? Get Clinical Tree
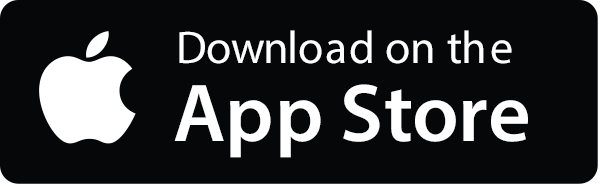
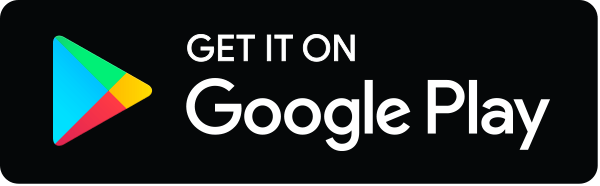