Chapter 5
Mechanical Ventilation in the ICU
Robert Chen and Jameel Ali
Chapter Overview
Mechanical ventilation is a frequent and very important part of management of the ICU patient. It is therefore essential that doctors working in this environment are familiar with the basics of mechanical ventilation. In this chapter, we discuss the function of the respiratory system and its relation to abnormalities requiring mechanical ventilatory support, the indications for mechanical ventilation, the setting up of the ventilator, monitoring the ventilated patient and the process of liberation from the ventilator.
Functions of the respiratory system and its malfunction leading to respiratory failure
The two main functions of the respiratory system are to eliminate carbon dioxide (ventilation) and to provide oxygenation. Respiratory failure may thus be classified into (1) Ventilatory or Hypercapnic Respiratory Failure and (2) Hypoxemic Respiratory failure.
Hypercapnic Respiratory failure results from: reduced respiratory drive from a variety of causes including drugs, chest wall abnormalities, neuromuscular disorders including muscular fatigue and other causes of increased work of breathing. The resulting increased alveolar pCO2 also leads to decreased alveolar pO2 and secondary hypoxemia.
Goals of Mechanical Ventilation
Based on the categorization of Respiratory failure, following are the goals of mechanical ventilation:
1.Decrease work of breathing
2.Improve gas exchange
3.Alleviate muscle fatigue
4.Allow time for lung healing in acute lung injury
5.Provide Hyperventilation — e.g., in head trauma
In overall management of the Respiratory failure the approach involves consideration of patient factors and those related to the ventilator.
Patient factors
The work of breathing should be decreased to the extent possible by:
1.Decreasing ventilatory demand-decrease CO2 production by decreasing carbohydrate and caloric load, decreasing dead space and maintaining oxygen supply, correcting metabolic acidosis and decreasing respiratory drive by reducing psychologic stress (including use of sedation);
2.Improving secretion clearance;
3.Reverse broncho spasm;
4.Optimize fluid balance by assessing for fluid overload, treating congestive heart failure including the use of diuretics where appropriate;
5.Reversing any correctible neuroendocrine-musculoskeletal abnormality, which may interfere with the mechanics of respiration;
6.Identifying and treating causes of increased pulmonary capillary permeability e.g., eradicating a septic focus.
Ventilator factors
This involves identifying factors that require ventilator assistance:
Mechanical ventilator assistance is required when the respiratory system can no longer maintain its normal function of providing adequate oxygen uptake and carbon dioxide clearance and this is manifested by:
1.Inability to maintain PaO2, PaCO2 and pH at acceptable levels (PaO2 <70 on FiO2 of 60%; PaO2/FiO2 <200, PaCO2 >55 torr and rising; pH 7.25 and lower.
2.Abnormal chest wall and/or lung compliance.
3.Inability to generate an adequate mechanical inspiratory force to sustain respiratory function (negative inspiratory force <15 cm H2O).
Clinical correlates of the above indications for institution of mechanical ventilation include: tachypnea, dyspnea, central cyanosis, hypertension, headache, sweating, tachycardia, irritability, confusion, loss of consciousness.
The Alveolar Gas Equation (Age) And Modes of Ventilation
Reviewing the AGE will facilitate understanding of the modes of ventilation and types of ventilators used:
PAO2 = (PAtm − PH2O)) × FiO2 − (PCO2/RQ),
PAO2 = alveolar oxygent tension
PAtm = atmospheric pressure
PH2O = pressure of water vapor (often assumed as 100% humidified)
FiO2 = fraction of inspired oxygen
PCO2 = pressure of alveolar carbon dioxide (CO2)
RQ = respiratory quotient (often assumed as 0.8).
whereas the pressure inside the circuit of a mechanically ventilated patient is often measured in centimetres of water (cm H2O), partial pressures of gasses are expressed in millimetres of mercury (mm Hg). Atmospheric pressure at sea level is considered to be 760 mmHg. As a standard, this also equals “zero” airway pressure as measured in cm H2O. Inspired air saturated with water vapour represents 47 mmHg of partial pressure displaced. By the time inspired air reaches the alveolus, it shares that volume (and thus partial pressure) with the CO2 continually transported by the alveolar capillaries. The RQ represents a “fudge factor” that allows peripherally measured arterial CO2 to better represent conditions within the lung. By substituting 0.21, or the fraction of atmospheric oxygen, the equation produces results nearing 100 mmHg for typical PCO2 values.
Recognizing that an airway pressure of zero refers to the pressure of 760 mmHg in the AGE opens up a new understanding of oxygenation. Increasing mean airway pressure (MAP) (i.e., 760 mmHg plus MAP) will result in increased PAO2. Increasing tidal volume delivered by a ventilator (thus airway pressure) increases MAP. Adding and increasing positive end expiratory pressure (PEEP) substantially increases MAP. Using pressure controlled (or pressure limited) ventilation (PCV) changes the shape of the inspiratory pressure curve such that a maximum pressure is maintained during the entire inspiratory phase. This further incrementally increases MAP. Changing the ratio of time spent in inspiration versus exhalation (I:E ratio) also significantly affects MAP. “Inverse ratio” ventilation, where more time is spent in the inhalation phase versus the exhalation phase further increases MAP. The ultimate version of prioritizing the inspiratory phase is airway pressure release ventilation (APRV). High airway pressures are maintained with only a brief moment where airway pressure is allowed to fall to allow for ventilation. Theoretically, if the patient never exhales such as the case with high frequency oscillation (HFO) or jet ventilation, the MAP is maximized.
Carbon dioxide removal from the alveolus serves as the other half of mechanical ventilation. pCO2 control can be summarized as related directly to minute ventilation.
Minute ventilation (V = Vt * RR),
Vt = tidal volume,
RR = respiratory rate.
Historically set tidal volumes and ventilation rates of 10–12 mL/kg × 10 breaths/minute in anaesthesia led to physiologic minute volumes but were found to be harmful in patients with lung injury.1 Such volumes may even have contributed to lung injury through mechanisms beyond barotraumas (i.e., pneumothorax).2 Current lung protective strategies in critical care suggest 6 mL/kg of ideal body weight as a starting point. Respiratory rates are increased as a consequence.
In the sickest patients, reducing the volume or pressure within the alveolus results in reduced lung injury through barotrauma and the volutrauma of surfactant insult with repeated alveolar collapse and distension.3 Prioritizing lower peak airway pressure and thus lower ventilating volume results in some hypoventilation. This strategy of permissive hypercapnia leads to a respiratory acidosis that is well tolerated.
In certain patients, pCO2 control will be more important. Avoidance of hypoventilation is the key in brain injury care. Many trauma patients present with concomitant CNS injury. Brain injury through congenital or acquired disease must be considered similarly. Other patients may tolerate acidosis poorly such as renal failure who might not tolerate pH related potassium shifts. Patients with sickle cell anaemia or pulmonary hypertension will also need to have good pCO2 control.
Related to pCO2 control is the titration of exhalation. As discussed earlier, oxygenation could be prioritized by increasing the percentage of the respiratory cycle spent in inhalation. Chronic lung disease, particularly of the obstructive variety is defined by elevated pCO2. Asthma, and other reversible reactive airways diseases are also marked by pCO2 elevation when in exacerbation. Those patients, by definition, have exhalation disease. Longer time spent in exhalation results in improved ventilation. Decreasing respiratory rate in special patients also increases time spent in exhalation.
Whereas the AGE predicts the theoretical pO2 generated by a ventilation strategy, the difference between the calculated pO2 and the measured pO2 is the alveolar–arterial gradient (A–a gradient). The normal gradient is in the 10–20 mmHg range. The PF ratio is calculated as PF = PaO2(mmHg)/FiO2 (as decimal). The PF ratio is a relative shortcut compared to the A–a gradient where a ratio of ≤200 defines adult respiratory distress syndrome (ARDS).4 Oxygenation failure is defined by an increased A–a gradient or abnormal PF rather than peripheral oxygen saturation while ventilated without consideration of FiO2.
Modes of Ventilation
There are two major categories of commonly used ventilators. Many modern ventilators are capable of delivering ventilation in both modes.
Volume Control (VC) ventilation is where rigidly timed mechanical breaths of set tidal volumes are delivered irrespective of the patient’s effort or respiratory phase. With obstructive processes or compliance changes, the set volume is still delivered but changes in airway pressure will result unless there is a feedback cut off maximum pressure alarm which inhibits further pressure increase and prompts intervention to protect from barotrauma.
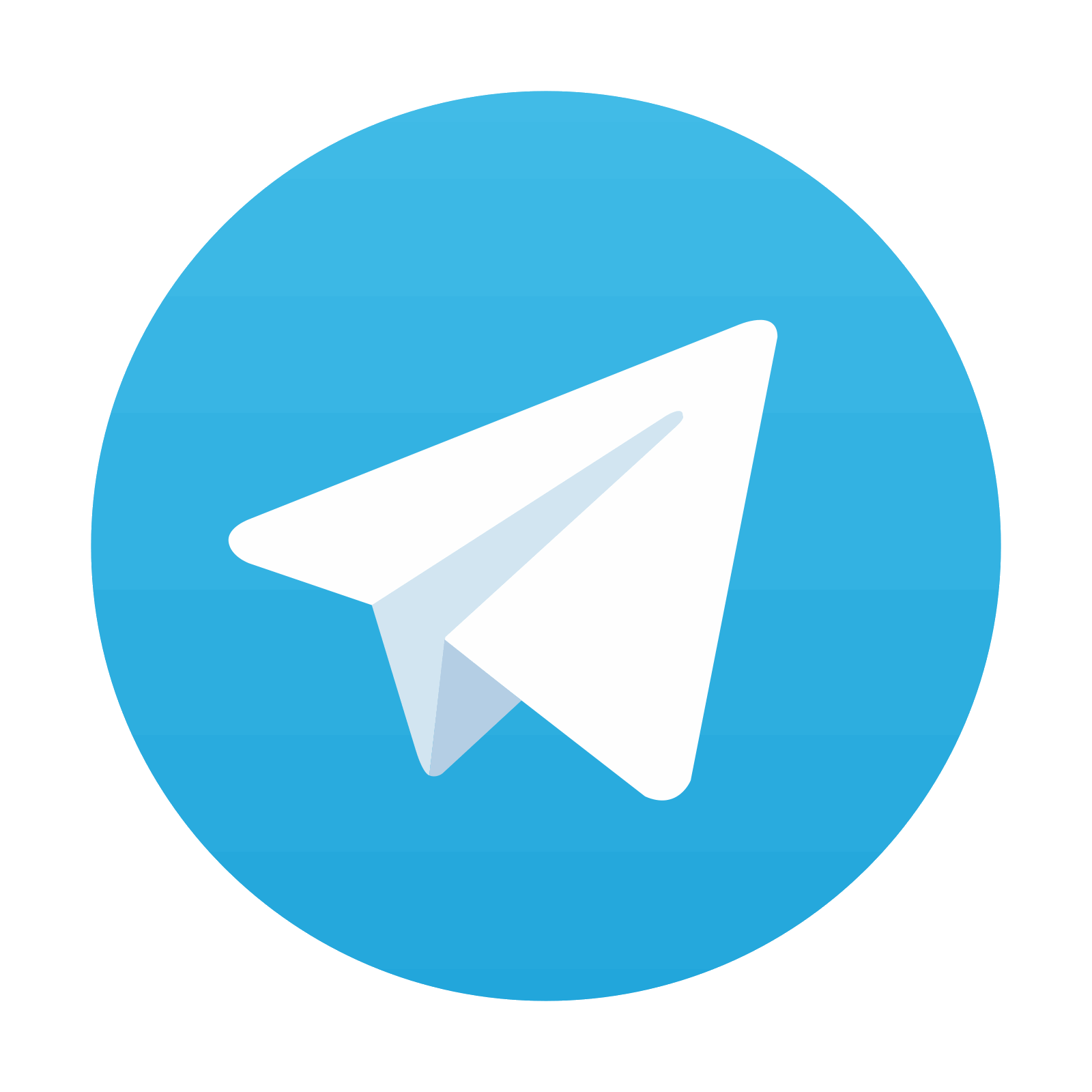
Stay updated, free articles. Join our Telegram channel
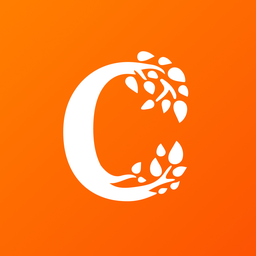
Full access? Get Clinical Tree
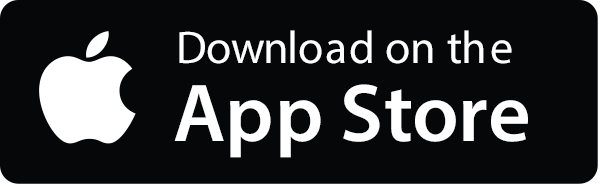
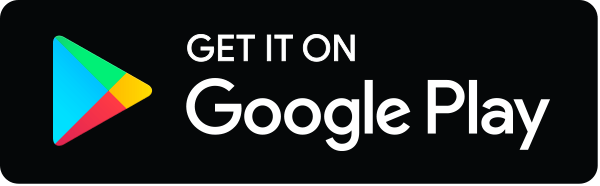