Abstract
Local anesthetics are a group of structurally related compounds which share as principal mechanism of action the blockade of voltage-gated sodium channels, resulting in reversible interruption of nerve signal transduction. Currently used local anesthetics are divided into amino amides, or amino esters. Each substance has distinct physicochemical properties, and local anesthetics can be administered continuously or together with adjuvants, allowing clinicians to tailor their anesthetic to procedure and patient. Next to sodium channel blockade, local anesthetics interact with other targets, for example calcium and potassium channels, and G-protein coupled receptors. The latter mode of action explains the anti-inflammatory properties of local anesthetics. Clinical application of existing local anesthetics, and development of novel local anesthetics, is hampered by systemic and local toxicity. Among the additives to local anesthetics, epinephrine is helpful in prolonging duration of action of medium-acting local anesthetics, and to reduce systemic absorption of any local anesthetic. Buprenorphine is an effective additive and has local anesthetic properties but causes excessive nausea and vomiting. Dexmedetomidine and clonidine are popular additives as well but can cause dose-dependent systemic side effects such as sedation, bradycardia and hypotension. Dexamethasone has the least systemic side effects, and the longest prolongation of nerve block duration. Emerging developments in the field of local anesthetics include the development of subtype-specific sodium channel blockers, cell type-specific or heat-assisted delivery, and various modes of encapsulation.
Keywords
local anesthetics, pharmacology, toxicology, pharmacokinetics, pharmacodynamics
Chapter Outline
Chemical Structure and Physicochemical Properties
Ester- Versus Amide-Type Local Anesthetics
Physiochemical Properties of Local Anesthetics: Clinical Implications
Electrophysiology of Neural Conduction
Voltage-Gated Na + Channels and Their Interaction With Local Anesthetics
Ultrasound and Intraneural Injection
Subtype-Specific Sodium Channel Blockers
Local Anesthetics and the Inflammatory Response
Historical Perspective
Cocaine, the first local anesthetic, was isolated from leaves of the coca plant, Erythroxylum coca, by Albert Niemann in 1860. The medicinal use of coca had long been a tradition of Andean cultures but it was only after chemical isolation that it became readily available in Europe. Its pharmacologic properties after oral ingestion were investigated and described by Sigmund Freud in his publication Über Coca in 1884. Freud’s descriptions of the numbing effect of cocaine on skin and mucous membranes prompted his Viennese colleague Carl Koller to use cocaine as a topical anesthetic for cataract surgery, which up until then was performed with the patient awake and associated with severe pain. Toward the end of the 19th century, cocaine was in widespread use for regional and local anesthesia for a myriad of medical indications, and it was also used as a food supplement—for example, in wine and soda beverages. However, the chemical purification of cocaine from coca leaves also increased its toxic and addictive properties, and in the early 20th century the need was identified to synthesize safer medical alternatives to cocaine.
Development of Modern Local Anesthetics
Both cocaine and the subsequent synthetic local anesthetics share as a basic property the reversible blockade of voltage-gated sodium channels. This resulted in two main clinical applications: (1) reversible interruption of nerve impulse propagation to achieve local or regional anesthesia, and (2) inhibition of cardiac ion channels as a class IB antiarrhythmic agent.
Using cocaine as the basic ester compound, the first derivative, procaine (also known by its trade name Novocaine), was synthesized in 1905. However, procaine was not the optimal local anesthetic because of its very long onset time, short duration of action, and low potency. A breakthrough came in the 1940s when lidocaine (Xylocaine) was introduced. Lidocaine was the first and a prototype compound of the amide class of local anesthetics, which are still in widespread use today. It had fewer undesirable effects than procaine and provided deeper anesthesia. Many new amide local anesthetics were subsequently introduced with differences in speed of onset, potency, and duration of action. Bupivacaine was synthesized in 1957 and rapidly gained popularity because of its long duration of action. However, because of the association of bupivacaine with cardiac toxicity, a less toxic long-acting drug was sought. Bupivacaine is a racemic mixture of the (+) and (−) enantiomers, with lesser toxicity associated with the S(−) form compared with the R(+) form. This led to the development of levobupivacaine and ropivacaine. Today many local anesthetic agents, including both ester and amide types, are available. Although they share the same basic structure, they have distinct physicochemical properties that allow clinicians to choose the optimal anesthetic for specific applications.
Chemical Structure and Physicochemical Properties
All currently available local anesthetics consist of a lipophilic phenyl ring and a hydrophilic tertiary amine joined by an intermediary ester- or amide-based linker ( Fig. 20.1 ).

Ester- Versus Amide-Type Local Anesthetics
Based on the nature of the intermediary chain, clinically used local anesthetics are classified as amino amides (e.g., lidocaine, prilocaine, bupivacaine) or amino esters (e.g., cocaine, procaine, chloroprocaine, tetracaine). Amide and ester local anesthetics differ in their chemical stability, metabolism, and allergic potential. Amides are extremely stable, whereas esters are relatively unstable, particularly in neutral or alkaline solution. Amide compounds undergo enzymatic degradation in the liver, whereas ester compounds are hydrolyzed in plasma by esterases. Cocaine, an ester, is an exception, as it is metabolized predominantly by the liver.
Allergy
True allergy to local anesthetics is rare but is thought to occur more commonly with ester local anesthetics than with amide local anesthetics. The accepted explanation is that metabolites of esters include p -aminobenzoic acid (PABA), which is immunogenic. Some preparations of amino amides contain methylparaben, which has a chemical structure similar to PABA and is a possible allergen in cases of allergic reaction to amide local anesthetics. Preservative-free preparations of local anesthetics are available to address the problems associated with methylparaben. Amide local anesthetics are considered to be one of the safest classes of drugs concerning allergy.
Chiral Forms
With the notable exception of lidocaine, the commonly used amide-type local anesthetics possess one chiral center in the amide piperidine ring. Therefore the plain solution of these substances is racemic, consisting of equal amounts of two possible stereoisomers, which are commonly designated on the basis of geometric configuration as levo (left) or dextro (right). These two forms can possess different pharmacologic properties that are of clinical importance. For example, bupivacaine is a racemic mixture, and levobupivacaine is the pure levorotatory enantiomer. Levobupivacaine demonstrates potency and efficacy comparable to bupivacaine but has significantly less cardiac and central nervous system (CNS) toxicity, likely related to reduced affinity for subtypes of sodium ion (Na + ) channels expressed in brain and cardiac tissues. Racemic- or R(+)-bupivacaine produces faster and more potent blockade of Na + channels in swine ventricular cardiomyocytes than levobupivacaine. In addition, racemic or R(+)-bupivacaine produces a greater reduction of the maximum rate of depolarization in animal cardiomyocytes, suggestive of the difference in Na + conductance.
Physiochemical Properties of Local Anesthetics: Clinical Implications
In general, with increasing length of the carbon backbone, local anesthetics exhibit greater lipid solubility, protein binding, potency, and duration of action. However, these relationships are not linear and are often influenced by multiple other factors. Local anesthetics must penetrate through the lipid-rich nerve sheaths and cell membrane to reach their targets, voltage-gated Na + channels (Na v ). The binding site of local anesthetics is located inside the channel pore and is not readily accessible from the extracellular side as inferred from pharmacologic studies. This concept has gained support from the atomic resolution structure of a bacterial voltage-gated Na + channel ( Fig. 20.2 ). Therefore local anesthetics must cross the nerve membrane into the cell interior by diffusing through the lipid bilayer to reach their binding site. Consequently, the potency of each local anesthetic is closely related to its lipid solubility and its dependence on pH.

The tertiary amine on the hydrocarbon backbone of local anesthetics is a weak base capable of accepting a hydrogen ion with low affinity to form a conjugated acid. Most local anesthetics have negative logarithm for the acid ionization constant (pKa) values relatively close to but higher than physiologic pH (with some exceptions such as prilocaine, which has a secondary amine and benzocaine that has a primary amine) ( Table 20.1 ). Around physiologic pH (7.4), local anesthetics exist in two forms, the positively charged acid and the neutral uncharged base form, with the respective ratio described by the Henderson-Hasselbalch equation:
pKa = log ( [ H + ] [ B − ] / [ BH ] ) pKa = pH + log ( [ B − ] / [ BH ] )
Local anesthetics cross the lipid membrane much faster in their neutral lipophilic form than their cationic form. Alkalinization by sodium bicarbonate addition to local anesthetics increases the pH and shifts the equilibrium in favor of the neutral base forms, which facilitates translocation of the local anesthetic into the cellular interior and speeds onset, but this is not advised for long-acting local anesthetics because of the risk of precipitation. Once inside the cell, the lower pH shifts the equilibrium toward the positively charged protonated form. The charged form antagonizes Na + channels more potently than the neutral form.
The onset of action of local anesthetic action depends on the route of administration and the dose or concentration of drug. In the subarachnoid space where the nerves lack a sheath, local anesthetics are able to reach their targets more readily, leading to a more rapid onset of nerve block with a much smaller dose compared with peripheral nerves. In peripheral nerve blocks, deposition of local anesthetic is in the vicinity of the nerves and the amount of drug that reaches the nerve depends on the diffusion of the drug and the proximity of the injection to the nerve. For a given route of administration, increasing the concentration can accelerate onset. For instance, chloroprocaine is much slower in onset than lidocaine at equal concentrations because its pKa of 9.1 favors the positively charged form at physiologic pH. However, a 3% solution is the typical concentration of chloroprocaine used clinically, which provides a much faster onset than other agents at their clinically used concentrations (e.g., 0.25% bupivacaine, 0.5% ropivacaine, or 1.5% mepivacaine) simply because there are more molecules present to diffuse into the nerve.
The duration of action of local anesthetics is determined primarily by their protein binding (see Table 20.1 ). Local anesthetics with a high affinity for protein remain bound to the nerve membrane longer. In other words, binding to Na + channels with higher affinity results in a channel blocking effect of longer duration. Duration of action is also influenced by the rate of vascular uptake of local anesthetic from the injection site. The duration of peripheral nerve blocks ranges from 30 to 60 minutes with short-acting agents such as procaine and chloroprocaine to nearly 10 hours with long-acting local anesthetics such as bupivacaine and tetracaine. The rate of vascular uptake significantly affects local anesthetic duration of action as local anesthetics provide their effect as long as they remain at the site of deposition. Therefore deposition of local anesthetics at a highly vascular site such as the intercostal space or inflamed tissue is associated with a higher rate of vascular uptake. Vasoconstriction slows the rate of vascular absorption and thus prolongs the duration of action. For this purpose, vasoconstrictive agents such as epinephrine and phenylephrine are frequently added to local anesthetics as adjuvants to increase duration. The prolongation of nerve block with vasoconstrictors is more prominent with local anesthetics of intermediate durations such as lidocaine and prilocaine than with longer-acting agents such as bupivacaine, possibly because the effect of long-acting local anesthetics outlasts that of vasoconstrictors. Local anesthetics themselves tend to have bimodal effects on vascular smooth muscle such that vasoconstriction results at lower doses, whereas vasodilation predominates at higher concentrations with some minor differences between individual agents.
Pharmacodynamics
Nerve Anatomy
The surface of the nerve axon is formed by the cell membrane consisting of a lipid bilayer that contains various proteins, including ion channels. Myelinated nerve axons are surrounded by myelin, produced by Schwann cells, that wraps around the axons to form the myelin sheath . When seen in longitudinal cross section, the myelin sheath is punctuated by gaps called nodes of Ranvier (see also Chapter 8 ). Other axons such as postganglionic autonomic efferent and some of the nociceptive afferent fibers lack a myelin sheath ( Fig. 20.3 ). Nerves are further organized within three layers of connective tissue: the endoneurium, perineurium, and epineurium (see Fig. 20.3 ). Nerve fibers are encased in endoneurium, loose connective tissue that consists of glial cells and fibroblasts along with blood capillaries. These fibers are grouped together into fascicles by dense collagenous perineurium. The fascicles are combined to form the peripheral nerve by a thicker layer of epineurium. The nerves are further encased in fascia. These are the structures that local anesthetics must penetrate to effectively block nerve conduction.

When looking at the sciatic nerve in the popliteal fossa as an example, three further peculiarities become evident. First, the share of nonneuronal tissue (connective tissue, fat, vessels) in peripheral nerves is high, at some locations more than half of the cross-sectional area. Second, while travelling distally, nerve fibers form a plexus in peripheral nerves. Third, before the sciatic nerve bifurcates into the tibial and common peroneal nerve, both nerves can be identified separately inside the thick connective perineurium of the sciatic nerve, at this point sometimes referred to as a paraneural sheath.
Nerves are typically characterized by their degree of myelination, axonal diameter, and speed of impulse conduction ( Table 20.2 ). They are classified as A, B, and C fibers, which roughly correspond to their decreasing cross-sectional diameters. A and B fibers are myelinated, and C fibers are unmyelinated. A and C fibers are further divided into subclasses by their anatomic location and physiologic functions.
Fiber | Diameter (µm) | Conduction Speed (m/s) | Sensitivity to Block | Myelination | Anatomic Location | Function |
---|---|---|---|---|---|---|
Aα | 15–20 | 80–120 | ++ | +++ | Afferent and efferent from muscles and joints | Motor, proprioception |
Aβ | 8–15 | 80–120 | ++ | +++ | Afferent and efferent from muscles and joints | Touch, pressure, proprioception |
Aγ, Aδ | 3–8 | 4–30 | +++ | ++ | Efferent to muscle spindles, sensory roots, and afferent peripheral nerves | Pain, temperature, touch/motor |
B | 4 | 10–15 | ++++ | + | Preganglionic sympathetic | Autonomic—preganglionic |
C | 1–2 | 1–2 | ++++ | − | Postganglionic sympathetic, sensory roots, and afferent peripheral nerves | Pain, temperature, touch |
Electrophysiology of Neural Conduction
The lipid bilayer of the axonal membrane is relatively impermeable to sodium ions but selectively permeable to potassium ions. The adenosine triphosphate (ATP)-dependent Na + /K + pump (sodium-potassium adenosine triphosphatase) exports Na + and imports potassium ions (K + ) in a 3 : 2 ratio to maintain a concentration gradient of these ions across the axonal membrane. The higher concentration of K + in the intracellular space and the greater permeability of the membrane to K + lead to the relative negative electrochemical potential of the cell interior. Resting neural membranes have an electrochemical potential of around −70 mV. When Na + channels open, sodium ions rush inward down the concentration gradient. Other channels, including Ca 2+ and K + channels, are sensitive to the change in electrical potential and open in response to the depolarization. Neurons are activated by the transduction of chemical, molecular, or thermal stimuli into electrical potential via the influx of cations to raise the electrical potential. The conduction of electrochemical impulses in axons is an all-or-none phenomenon. When the depolarization is strong enough, the stimulus is conducted by sequential depolarization of the neural membrane along the axonal length via the opening of Na + channels and net inward movement of sodium ions. The sodium ions inside the cell diffuse along the axon in both directions and passively depolarize the adjacent membrane, thereby triggering the opening of additional Na + channels by reaching their activation threshold potential. Because the upstream region of the membrane is already depolarized and in a refractory state, the electrical impulse can propagate only in an anterograde direction along the axon ( Fig. 20.4A ). In myelinated axons, the myelin sheath serves as insulation, and this local phenomenon takes place only at the nodes of Ranvier. Therefore the nerve impulse is able to “skip” the length of myelin to the next node (saltatory conduction), making much faster conduction possible (see Fig. 20.4B ). As a rule of thumb, three nodes of Ranvier need to be blocked to reliably interrupt impulse propagation.

Voltage-Gated Na + Channels and Their Interaction With Local Anesthetics
Ion channels are multi-subunit transmembrane proteins that fold in a complex manner to form ion selective pores gated by voltage or ligands (see Fig. 20.2 ). Ion channels can switch between different conformations in a voltage-dependent manner that determines pore opening (activation), closing (inactivation), and reactivation (to the resting state) ( Fig. 20.5 ). Voltage-gated Na + channels consist of a single α subunit and varying auxiliary β subunits. The α subunit forms the ion-conducting pore of the channel and consists of four homologous domains, each with six α-helical transmembrane segments ( Fig. 20.6 ). The loops that connect the S5 and S6 segments of these α helices of each of the four domains are positioned extracellularly and extend inward, which form the narrowest point of the channel pore and are thought to provide its ion selectivity (see Fig. 20.2 ). In the resting state, the ion pore of the Na + channel is closed (see Fig. 20.5A ). Depolarizing voltage changes lead to movement of the voltage sensor (S1-S4) as the result of outward movement of positive charges in the S4 segment, which in turn leads to the rearrangement of S6 segments that results in opening of the channel pore (see Fig. 20.5B ). The activated channel is inactivated within milliseconds by another conformational change, resulting in the movement of the S6 segment and the S5-S6 linker acting as an inactivation gate (see Fig. 20.5D ).


The inactivated state differs from the resting state in its molecular conformation as well as its interaction with local anesthetics, which selectively bind and stabilize the inactivated state. Local anesthetics dose-dependently decrease peak Na + current through voltage-gated Na + channels. The binding site for local anesthetics is located interior to the ion-selective pore and can be approached via two different pathways: diffusion of the uncharged form through the cell membrane followed by access to the binding site from the cytosolic side (hydrophilic pathway), and laterally through the lipid membrane (hydrophobic pathway) (see Fig. 20.2B ). When bound, local anesthetics stabilize the inactivated state and prevent further activation. They can also bind in the ion channel pore to prevent ion flux (open-state block). Local anesthetics have a higher affinity for Na + channels in the activated (open) and inactivated (channel pore in open conformation but intracellularly closed by inactivation gate) states than those in the resting closed state (see Fig. 20.5C and D ). The difference in the affinity is attributed to the difference in the availability of the two pathways for local anesthetics to reach their binding site. The binding site is more accessible in the activated state than in the resting state.
In the presence of local anesthetics, repeated depolarization results in an incremental decrease in Na + current until it reaches a newer steady level of inhibition, which is termed use-dependent or phasic inhibition . With repeated depolarization, a greater number of Na + channels are in the active or inactivated state and are blocked by local anesthetics. Furthermore, the dissociation rate of local anesthetics from their binding site is slower than the rate of transition from inactivated to resting state. Therefore repeated stimulation results in accumulation of local anesthetic–bound Na + channels, which manifests as use-dependent inhibition or block ( Fig. 20.7 ). The positive charge introduced by the charged local anesthetic is thought to play a minor role.

Na + Channel Diversity
There are multiple subtypes of voltage-gated Na + channels arising from variation in the homologous α-subunit genes. The 10 subtypes now known in mammals (Na v 1.1 through 1.9 and Na x ) are differentially expressed in various tissues with cell- and tissue-specific functions. Sodium channels such as Na v 1.8, Na v 1.9, and Na v 1.7 are expressed exclusively in peripheral neurons. The Na v 1.7 subtype determines the ability of nociceptive neurons to transmit noxious stimuli. Rare loss-of-function mutations of Na v 1.7 are reported in patients with channelopathy-associated insensitivity to pain, in which patients have selective lack of pain and smell sensation. On the other hand, several gain-of-function mutations of genes related to the regulation or function of Na v 1.7 result in overactivity of this channel and are associated with the congenital pain disorders (erythermalgia, also termed erythromelalgia ) and paroxysmal extreme pain disorder. Na v 1.7 also appears to be involved in the development of inflammatory pain.
Mechanism of Nerve Block
It was assumed that only a very small fraction (1%-2%) of local anesthetic reaches the nerve membrane even when placed in close proximity to the nerve. With the advent of ultrasound-guided targeting, this fraction might be higher, but still a considerable amount of anesthetic is lost along the concentration gradient across different tissues. The quality of nerve blockade is determined by the potency, concentration, and volume of the local anesthetic. The potency of a local anesthetic can be expressed as the minimum local anesthetic concentration at which complete nerve block is established. The volume of local anesthetic is also important as a sufficient length of axon must be blocked to prevent regeneration of the impulse in the adjacent node of Ranvier. This is understood by the phenomenon of decremental conduction, whereby depolarization of the membrane decays with the distance away from the front of the action potential, and impulse propagation stops when the depolarization falls below the conduction threshold ( Fig. 20.8 ). If less than the critical length of the axon is blocked (usually assumed to be three nodes of Ranvier), the action potential can be regenerated in the proximal membrane segment or node when the decaying depolarization is still above threshold for Na + channel activation.
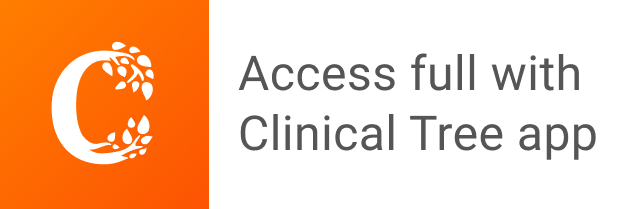