Chapter 10
Local Anesthetics
Anatomy of the Peripheral Nerve
Between Schwann cells are periodic segments of nerve that do not contain myelin. These areas, known as nodes of Ranvier, are where conduction is propagated. Voltage-gated sodium channels (Nav) are located in these nonmyelinated segments and are the primary site at which local anesthetics exert their action. Action potentials jump from node to node, and this phenomenon is known as saltatory conduction, which significantly facilitates conduction speed along the axon.1,2 Myelinated nerves are larger, conduct impulses faster, and are more difficult to block with local anesthetics than are unmyelinated nerves3,4 (Figure 10-1).
Peripheral nerves have structures containing bundles of axons called fasciculi. Three layers of connective tissue—the endoneurium, perineurium, and epineurium—also are components of the peripheral nerve.4,5 The endoneurium, which is a delicate connective tissue composed of longitudinally arranged collagen, surrounds and embeds the axons in the fasciculi. The perineurium, which consists of layers of flattened, overlapping cells, binds a group of fascicles together. The epineurium, which surrounds the perineurium, is composed of areolar connective tissue that functionally holds the fascicles together to form the peripheral nerve.5 These layers of connective tissue are important because they serve as barriers through which local anesthetics must diffuse if they are to exert their pharmacologic action (Figure 10-2).
Neuron Electrophysiology and the Action Mechanism of Local Anesthetics
Measurement with an electrode placed in the axoplasm of a resting peripheral nerve demonstrates a negative membrane potential of −70 to −90 mV.3,5 This voltage difference across the neuronal membrane at steady state is called the resting membrane potential (Figure 10-3). An ionic imbalance between the axoplasm and the extracellular fluid causes the electrical potential. Several physiologic mechanisms create the ionic gradient; the primary one is an active, energy-dependent process executed by a sodium-potassium pump (Na+-K+/ATPase) located in the axolemma.6,7
Although the membrane is relatively permeable to the outward diffusion of K+, an intracellular-to-extracellular K+ ratio of 150:5 mmol, or 30:1, exists. An important contributor to this concentration difference is the impermeability of the membrane to other cotransported ions such as Na+.7 In addition, the movement of K+ out of the neuron leaves an excess of intracellular negatively charged organic ions. The negative charge results in an electrostatic counterforce that limits K+ movement out of the neuron.
Two opposing forces influence K+ movement into and out of the neuron. First, a concentration gradient pushes K+ outward. Second, an electrostatic gradient, created by the impermeability of the membrane to cations, tends to keep the K+ in the cell. The net effect of these counterforces is modest movement of K+ out of the cell, and this movement creates an intracellular negative charge. The Nernst equation expresses the charge created by the K+ concentration gradient3:
Determination of the resting membrane potential is not as simple as the Nernst equation for K+ indicates, because Na+ and chloride (Cl−) ions have a minor role in establishing the intracellular resting potential.3
When an electrical impulse is applied to a resting nerve, the membrane potential is reversed because of the intracellular movement of Na+. This occurs because of the higher concentration of Na+ outside the cell and the stimulation-induced increase in membrane permeability to this ion. The sudden influx of Na+ that occurs in response to stimulation overrides the efflux of K+ directed at maintaining the resting membrane potential. Once the process has reversed the membrane potential to 20 mV, an outward electrochemical gradient develops; this gradient resists the concentration-dependent, inward diffusion of Na+.5 This state of equilibrium causes the Na+ channels to close. Shortly after Na+ enters the cell, K+ channels begin to open, and the ion rapidly diffuses out of the neuron, according to its concentration gradient. The active removal of intracellular Na+ by the Na+-K+ pump and the passive diffusion of K+ outward restore the resting membrane potential. During repolarization, three Na+ ions leave the cell for each two K+ ions that enter8 (Figure 10-4).
The sequence of events that results in an action potential results from the passage of ions through pores, or “channels,” located in the axolemma. These channels, which are composed of globular proteins, have transmural orientation to the phospholipid molecules that constitute the axolemma.9 Although K+ and calcium (Ca2+) channels are important, the Na+ channels are the most significant and best understood with respect to the initiation and propagation of the action potential.3,10–14
Mechanism of Action
As noted earlier, local anesthetics work by reversibly binding to the voltage-gated sodium channels (Nav ). Sodium channels have three functional states: resting (closed), open, and inactive. The resting state exists when the membrane is at its resting potential. When a nerve is stimulated, reversal of the membrane potential occurs until the threshold potential is reached. When this happens, a conformational change in the proteins that compose the channel occurs resulting in the open state. An inactive state, characterized by the return of the Na+ channel to an impermeable state, follows the open state. This state, which prevents initiation of an action potential, lasts until the restoration of the resting membrane potential.10 This three-state concept describes the changes in the Nav that occur during depolarization and repolarization. Local anesthetics preferentially bind to both the open or inactivated states and not to the closed state. This is referred to as the guarded receptor or modulated receptor hypothesis of local anesthetic action. The open or inactive state may increase the affinity for binding, the physical access of the drug to the receptor, or both.11,15 In addition, it has long been noted that local anesthetics work faster as the Nav is repetitively depolarized. This is termed as use-dependent or phasic block. The more frequently the channel is depolarized, the more time it is available in the open and inactive states and thus available to local anesthetic blockade16 (Figure 10-5).
Local anesthetics block the propagation of the action potential by binding reversibly to specific receptors within or adjacent to the internal opening of the Nav channel.17 Studies have indicated that these receptors, located on the intracellular side of the cell membrane, have a greater affinity for the charged or ionized form of the local anesthetics.8,10,18 The uncharged or nonionized portion of the local anesthetic must first penetrate the cell membrane entering the axoplasm before they produce their effects. Figure 10-6 shows the penetration of local anesthetic into a nerve and subsequent access to the receptor. The sequence is as follows:
1. Almost all clinically useful local anesthetics (except benzocaine) are tertiary amines and when injected, will exist in both nonionized (lipid soluble) and ionized (water soluble) forms according to their particular pKa (negative logarithm of the acid ionization constant) and the pH of the tissue or compartment they are in.
2. Local anesthetics must gain access to the interior of the neuron to reach their receptor. This occurs by the diffusion of the lipid soluble nonionized fraction across the cell membrane.
3. Once inside the neuron, a new equilibrium forms between ionized and nonionized fractions. The ionized fraction binds to the receptor on the inside of the Nav.
Benzocaine is a secondary amine and thus is permanently nonionized or neutral. It penetrates the lipid bilayer and can directly inhibit the Nav without entering the axoplasm first.12
Local anesthetics have additional effects on G-protein–coupled receptors affecting intracellular calcium signaling pathways. The inflammatory modulating action of local anesthetics may result from interruptions in these pathways. The antiinflammatory response to local anesthetics also occurs due to suppression of polymorphonuclear leukocyte priming, which prevents overactive inflammatory responses without impairing host defenses or suppressing normal inflammation.16,19
It must be noted that many questions remain as to the mechanism of the clinical effects of local anesthetics in different types of nerve blocks. Different receptors may be involved in their action in peripheral nerves as opposed to spinal or epidural effects.20
Nerve Fiber Sensitivity and Differential Block
It has been observed that nerves functionally have different sensitivity or rates of effect when exposed to local anesthetics. For example, in most major nerve blocks, loss of autonomic function occurs first, followed in sequence by perception of superficial pain, touch, and temperature, motor function, and proprioception.21 This phenomenon is termed differential block. Seen clinically, an excellent example of differential block occurs with the use of bupivacaine. When administered epidurally for labor pain, this local anesthetic spares motor function while providing adequate analgesia.22,23
Essential to the understanding of differential block is the concept that the diameter and myelination of nerve fibers influence the sensitivity to local anesthetics. For simplicity, nerve fibers are separated into three groups—A, B, and C—on the basis of diameter.24,25
The A fibers are further divided into four subgroups known as alpha, beta, gamma, and delta fibers. The alpha fibers are the largest in diameter (12 to 20 µm) and the most heavily myelinated; they have the fastest conduction velocity of all the fibers, including B and C fibers. Alpha fibers are responsible for motor functions and proprioception. The A beta (5-12 µm) and A gamma fibers (3-6 µm) have conduction velocities second only to A alpha fibers. The A beta fibers provide motor function, touch, and pressure sensation; the A gamma fibers innervate muscle spindles and are responsible for reflexes. The A delta fibers provide pain and temperature sensation. These fibers have a smaller diameter (1-5 µm) and slower conduction velocity than other A fibers. The beta, gamma, and delta fibers are all myelinated to a similar extent.26
B fibers have a similar diameter (less than 3 µm) to A delta fibers; however, they exhibit slower conduction velocity and less myelination than the A fibers. These fibers constitute the preganglionic autonomic nerves. The C fibers, which conduct pain and temperature impulses, are the smallest of all fibers (0.3-1.3 µm) and have the slowest speed of conduction. These are the only fibers that are unmyelinated.8 Nerve fiber characteristics and local anesthetic sensitivity are summarized in Table 10-1.
It was first believed that nerve fiber diameter was the sole determinant of differential blockade.27 This assumption came from the results of isolated in vitro studies performed only on myelinated nerves. Subsequent isolated studies on the small, unmyelinated C fibers revealed that they were more resistant to blockade than the larger A delta or B fibers.8
This apparent inconsistency can possibly be explained by the concept of conduction safety. This concept refers to the voltage change needed for the propagation of the action potential along the nerve. This voltage change is significantly greater than the action potential threshold and provides a safety factor for impulse conduction. Gissen et al.28 defined this safety factor as the “ratio between the magnitude of the action potential and the magnitude of the critical membrane potential.” Research has indicated that the margin of safety for transmission is greater in small, slow fibers than in large, fast fibers.
Lastly, differential block may be influenced by the rate of diffusion of local anesthetic molecules across multilayered lipoprotein membranes of the myelin sheath. For example, the clinical resistance to blockade observed in A fibers may be the result of a slower onset resulting from a greater diffusion barrier. As discussed in more detail later, diffusion can be influenced by such factors as the pKa and the concentration of the local anesthetic, as well as the pH of the surrounding tissue and nerve fiber.28
As the preceding discussion indicates, the concept of differential block is more complex than originally proposed. Future research will probably indicate that an isolated mechanism does not explain this phenomenon, but rather several factors interact to produce the effect.22
Chemical Structure of Local Anesthetics
The local anesthetics used clinically for neural blockade are aminoesters or aminoamides and are similar in chemical structure. In general, these drugs have three characteristic segments: (1) an intermediate ester or amide carbon group separates (2) an unsaturated (aromatic) ring system from (3) an amine end (Figure 10-7). The aromatic ring provides lipophilic characteristics, whereas the tertiary/quaternary amine gives hydrophilicity to the molecule. The amine portion is able to become ionized in physiologic pH and thus hydrophilic.
Chemically, the major difference among local anesthetics is in their ester or an amide linkage that binds the aromatic ring to the amine group. This linkage is responsible for the classification of these drugs as either esters or amides. The type of linkage is important clinically because it has implications for metabolism, duration, and allergic potential (Figure 10-8). Box 10-1 lists the local anesthetics according to chemical class. The chemical structures are important in determining the pharmacologic effects of these drugs. Minor chemical alterations to drugs within these two “bond-related” groups can result in significant changes in drug potency, speed of onset, and duration of action and potential for producing differential block (Figure 10-9).5,16,29 These changes are discussed in detail as the specific pharmacologic factors associated with local anesthetics are noted. Table 10-2 summarizes the clinical differences between the ester and amide local anesthetics.
TABLE 10-2
Clinical Differences Between the Ester and Amide Type Local Anesthetics
Esters | Amides |
Ester metabolism is catalyzed by plasma and tissue cholinesterase via hydrolysis; occurs throughout the body and is rapid | Amides are metabolized in the liver by CYP1A2 and CYP3A4 and thus a significant blood level may develop with rapid absorption |
Although local anesthetic allergy is uncommon, esters have a higher allergy potential, and if patients exhibit an allergy to any ester drug, all other esters should be avoided | Allergy to amides is extremely rare; there is no cross allergy among the amide class or between the ester and amide agents |
Ester drugs tend to be shorter acting due to ready metabolism; tetracaine is the longest acting ester | Amides are longer acting because they are more lipophilic and protein bound and require transport to the liver for metabolism |
Pharmacodynamics and Pharmacokinetic Concepts
An important difference to note when describing the pharmacokinetics of local anesthetics is intuitive yet bears discussion. Unlike most medications, these agents are meant to remain localized in the area of injection or application. The higher the concentration (number of molecules) of drug injected that remain in the area of the nerve or nerves to be blocked, the faster the onset of action. If multiple nerves are being blocked, a greater intensity may also be evident. Therefore, systemic absorption away from the deposition site results in the offset and termination of drug effect, rather than the onset as with most other drugs. Factors that affect absorption, such as the vascularity and blood flow of the injection area, lipid and protein binding, and addition of vasoconstrictors, greatly influence duration of action. The same local anesthetic dose and concentration injected in different areas of the body, with or without added epinephrine, can result in vastly different durations of action. Absorption also influences toxicity. The slower a local anesthetic is systemically absorbed, the less likely that high blood levels and therefore central nervous system (CNS) or cardiac toxicity will result. Drug metabolism and elimination more readily “keep up” with absorption, ensuring that toxic blood levels are avoided. A conceptual kinetic depiction of the fate of an injected local anesthetic is given in Figure 10-10.
Potency
There is a strong relationship between the lipid solubility of local anesthetics and their potency.28,29 This finding is understandable, considering that the axolemma and myelin sheath are composed primarily of lipids29,30; therefore, lipid-soluble drugs pass more readily through the nerve membrane. Larger, more lipid-soluble local anesthetics are relatively water insoluble, highly protein bound, and less readily washed out from nerves and surrounding tissues. They bind to Nav channels with a higher affinity than agents with lower lipid solubility. Increased lipid solubility correlates with increased protein binding, increased potency, longer duration of action, and a higher tendency for severe cardiac toxicity.12,29 It follows that fewer molecules or lower concentrations of these drugs are required for the production of blockade than if non–lipid-soluble anesthetics are used.31 Changes in either the aromatic or amine moieties of the local anesthetic molecule can affect the lipid-water partition coefficient. In the amide series, for example, the addition of a butyl group to the amine end of mepivacaine leads to the formation of bupivacaine. Bupivacaine is 26-fold as lipid soluble and fourfold as potent as mepivacaine. In the case of the esters, the addition of a butyl group to the aromatic end of procaine produces tetracaine, which is considerably more lipid soluble and potent than procaine.16
Factors other than lipid solubility can affect potency. For example, the potency of local anesthetics as demonstrated in isolated in vitro studies is not always the same as that observed in vivo. The discrepancy between in vitro and in vivo findings may be the result of many factors, including the vascular and tissue distribution properties of the drug.32 For example, the results of in vitro studies show that lidocaine is twice as potent as prilocaine; however, clinical use indicates that they have similar potencies. This may be explained by the fact that lidocaine has greater vasodilating properties than prilocaine and therefore may be absorbed away more rapidly, leaving less drug available for interaction with the neuron.33–35
Duration of Action
The duration of action of local anesthetics demonstrates a relationship to protein binding and lipid solubility.29,36 In theory, drugs that have a high affinity for protein and lipids attach more firmly to these substances in the vicinity of the Nav channel receptor. This means that the drug remains in the channel and surrounding areas for a longer time, producing prolonged conduction blockade.29
It appears therefore that there is a correlation between the degree of protein binding and duration of the local anesthetic. The addition of larger chemical radicals to the amide or aromatic end of the drugs results in greater protein binding. The duration is directly proportional to plasma protein binding, presumably because the local anesthetic receptor on the neural membrane is also composed of protein.3,5,37 It has been posited that local anesthetics that have increased protein-binding properties (e.g., ropivacaine 94%, bupivacaine 97%) produce longer-duration anesthesia as a consequence of more efficient binding of the anesthetic to the Nav channel.38 For example, bupivacaine is more than 90% bound to plasma protein; however, its homologue, mepivacaine, is only 65% bound.36 The duration of action of bupivacaine is significantly longer than for mepivacaine. Local anesthetics are weak bases and bind mainly to α1-acid glycoprotein (AAG). Secondary binding to albumin also occurs.
Onset of Action
As stated previously, local anesthetics must diffuse through the axolemma before they can interact with receptors. How readily they diffuse through the nerve membrane depends on their chemical structure, lipid solubility, and state of ionization. Of these, ionization is the most important, because the charged or ionized form of a drug does not penetrate membranes well.30,32,37 In other words, the more ionized a local anesthetic, the slower it will penetrate a nerve.
Local anesthetics are bases. The pKa of a drug is the pH at which 50% of the drug is in the charged, or ionized, and water soluble form while the remaining half is uncharged, or nonionized, and lipid soluble. A basic drug becomes predominantly ionized if it is placed in an environment with a pH that is significantly less than its pKa. Therefore drugs that have a greater pKa are ionized to a greater extent at body pH than those with a lower pKa. For example, if lidocaine (pKa 7.74) is placed in plasma (pH 7.4), 65% of the drug is ionized and 35% remains un-ionized. Similarly, if tetracaine (pKa 8.6) is placed in plasma, 95% of the drug becomes ionized and 5% remains un-ionized.29
Because their ionization is less, local anesthetics with lower pKa (7.6 to 7.8), such as lidocaine, mepivacaine, and prilocaine tend to have a more rapid onset of action than drugs with a greater pKa (8.1 to 8.6), such as bupivacaine, tetracaine, and procaine. Chloroprocaine is one exception; it has a high pKa but retains a rapid onset, probably because of the clinical use of high concentrations of the drug, which attenuates the ionization effect. In general, the closer the pKa is to pH 7.4, the more rapid the onset. Some researchers downplay the role of pKa on onset.29
The classification presented in Table 10-3 assists in the selection of an appropriate drug with respect to pharmacokinetic properties.39
Vasomotor Action and Absorption
All local anesthetics except cocaine, ropivacaine, and lidocaine produce relaxation of vascular smooth muscle.33,40 The resultant vasodilation increases blood flow to the tissue in which the drug is deposited. This results in an increase in the drug’s absorption, which limits its duration of action and increases the probability of toxic effects. It is interesting to note that ropivacaine and lidocaine are the only parenterally administered local anesthetics with mild vasoconstrictive properties.40 Cocaine also has vasoconstrictive properties because of its ability to block reuptake of norepinephrine. It is used only topically.
All local anesthetics are not affected equally when epinephrine is added to the solution. There is a definite benefit in extending the duration of analgesic effects with both short- and intermediate-acting agents. The prolongation of the duration with long-acting drugs is less well defined.40
The speed of absorption and entry of the local anesthetic into the systemic circulation obviously has significant implications for toxicity. Absorption of drugs generally occurs in the following order of rapidity: interpleural blocks > intercostal > caudal > epidural > brachial plexus > sciatic-femoral and subcutaneous blocks.16 Thus blood levels measured after an interpleural block will be higher with a greater potential for toxicity than with a subcutaneous block.
The total dose of local anesthetic, rather than the volume or concentration, linearly determines the peak plasma concentration.41 For example, 400 mg of lidocaine yields the same peak plasma concentration regardless of whether 40 mL of a 1% or 80 mL of a 0.5% is injected.
Local anesthetic additives include α2-adrenergic agonists, opioids, sodium bicarbonate, ketorolac, and hyaluronidase. These are variously added to increase the safety, quality, intensity, duration, and rate of onset of anesthesia, as well as reduce blood loss. Alpha2-adrenergic agonists such as clonidine and dexmedetomidine have local anesthetic properties and can alter the nerve block characteristics.42,43 The addition of 100 mcg of clonidine to a local anesthetic solution prolongs the duration of the long-acting agents approximately 100 additional minutes with minimal side effects. The effect is produced by inhibition of the hyperpolarization-activated cation current (Ih current). This current normally restores nerves from the hyperpolarized state to resting potential. The effect is more pronounced in C-fibers (sensory) than Aδ (motor). That makes the effects mostly sensory specific. Cost has limited the routine use of clonidine.40
The addition of a vasoconstrictor (e.g., epinephrine) to local anesthetics can reduce the rate of vascular absorption, allowing more of the drug to stay in the local area where it was injected. The availability of the drug for neuronal uptake is increased, resulting in a longer and more profound block. Of importance, the slower rate of absorption also attenuates the peak plasma concentration of the drug, thereby reducing systemic toxicity. The magnitude of this effect depends on the drug, dose, and concentration of both the local anesthetic and the vasoconstrictor, as well as the site of injection.7 For example, addition of epinephrine to mepivacaine prolongs the time to maximum arterial plasma drug concentration in all situations; however, adding epinephrine to a 2% solution used for an intercostal block has the greatest effect.44
Epinephrine does not prolong the duration of blockade to the same extent with all local anesthetics. For example, it prolongs the duration for local infiltration, peripheral nerve block, and epidural anesthesia with procaine, mepivacaine, and lidocaine.30,45–48 Research indicates that adding epinephrine to lidocaine solutions increases the intensity and duration of block.48 The early increase in intensity is not matched with an increase in intraneural lidocaine content at these early times, although the prolonged duration of block by epinephrine appears to correspond to an enlarged lidocaine content in a nerve at later times, as if a very slowly emptying “effector compartment” received a larger share of the dose. The increase in early analgesia without increased lidocaine content may be explained by a pharmacodynamic action of epinephrine that transiently enhances potency of lidocaine, but also by a pharmacokinetic effect that alters the distribution of the same net content of lidocaine within the nerve. In the case of prilocaine and bupivacaine, infiltration and peripheral nerve blocks are prolonged with epinephrine, whereas no significant effect occurs with epidural anesthesia.49 The rationale for this discrepancy might be that epidural fat significantly absorbs ropivacaine and bupivacaine because of their high lipid solubility. These drugs are released slowly from the fat deposit, which could prolong the block.47,49–51 This process overrides the effects of epinephrine on duration of action. In addition, the drug concentration can contribute to the differential effect seen with epinephrine. For example, epinephrine can prolong epidural blocks with 0.125% or 0.25% bupivacaine when used in patients in labor.50,51 Conversely, epinephrine has less effect with the epidural administration of 0.5% or 0.75% bupivacaine.52
The addition of epinephrine does not attenuate the peak plasma level of all local anesthetics; for example, epinephrine significantly reduces the peak plasma concentration of lidocaine and mepivacaine, regardless of the site of administration. On the other hand, epinephrine does not significantly affect the peak plasma level of prilocaine or bupivacaine after epidural anesthesia. The lack of effect seen with prilocaine may be explained by its slower absorption and rapid tissue redistribution. In the case of bupivacaine, it may be explained by the significant lipid solubility and uptake in the epidural adipose tissue.28 There is some controversy as to whether epinephrine may produce neurotoxicity when added to local anesthetics. Some clinicians only recommend its use for nerve blocks done without ultrasound guidance or where the needle tip and local anesthetic spread are not adequately visualized as a safety measure to detect intravascular injection.40
Studies that have compared vasoconstrictors conclude that epinephrine is superior to drugs such as phenylephrine and norepinephrine in producing vasoconstriction with local anesthetics.53,54 The usual concentration of epinephrine used for this purpose is 1:200,000 or 5 mcg/mL.
Miscellaneous Factors That Influence Onset and Duration
Local anesthetics are basic drugs. As discussed previously, they have both water- and lipid-soluble properties. Factors that raise the pH of their environment increase their lipid solubility, and, conversely, lower pH environments result in increased water solubility. These changes to pH result in altered proportions of lipid- and water-soluble fractions of the drugs, which may have clinical consequences. At times, the term used for this phenomenon is ion trapping. Ion trapping results from changes in pH in relationship to the pKa of the agent. Instances in which ion trapping may have clinical consequences are noted in Box 10-2.
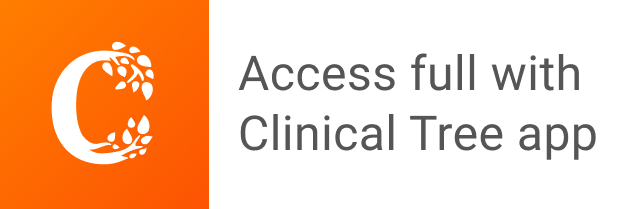