
Local anesthetics are poorly soluble in water and therefore are marketed most often as water-soluble hydrochloride salts. These hydrochloride salt solutions are acidic (pH 6), contributing to the stability of the local anesthetic. An acidic pH is also important if epinephrine is present in the local anesthetic solution, because this catecholamine is unstable at an alkaline pH. Sodium bisulfite, which is strongly acidic, may be added to commercially prepared local anesthetic–epinephrine solutions (pH 4) to prevent oxidative decomposition of epinephrine.
Structure–Activity Relationships
Modifying the chemical structure of a local anesthetic alters its pharmacologic effects. For example, lengthening the connecting hydrocarbon chain or increasing the number of carbon atoms on the tertiary amine or aromatic ring often results in a local anesthetic with a different lipid solubility, potency, rate of metabolism, and duration of action (Table 10-1). Substituting a butyl group for the amine group on the benzene ring of procaine results in tetracaine. Compared with procaine, tetracaine is more lipid soluble, is 10 times more potent, and has a longer duration of action corresponding to a 4- to 5-fold decrease in the rate of metabolism. Halogenation of procaine to chloroprocaine results in a 3- to 4-fold increase in the hydrolysis rate of chloroprocaine by plasma cholinesterase. This rapid hydrolysis rate of chloroprocaine limits the duration of action and systemic toxicity of this local anesthetic. Etidocaine resembles lidocaine, but substituting a propyl group for an ethyl group at the amine end and adding an ethyl group on the alpha carbon of the connecting hydrocarbon chain produces a 50-fold increase in lipid solubility and a 2- to 3-fold increase in the duration of action.

Mepivacaine, bupivacaine, and ropivacaine are characterized as pipecoloxylidides (see Fig. 10-2). Mepivacaine has a methyl group on the piperidine nitrogen atom (amine end) of the molecule. Addition of a butyl group to the piperidine nitrogen of mepivacaine results in bupivacaine, which is 35 times more lipid soluble and has a potency and duration of action 3 to 4 times that of mepivacaine. Ropivacaine structurally resembles bupivacaine and mepivacaine, with a propyl group on the piperidine nitrogen atom of the molecule.
Racemic Mixtures or Pure Isomers
The pipecoloxylidide local anesthetics (mepivacaine, bupivacaine, ropivacaine, levobupivacaine) are chiral drugs because their molecules possess an asymmetric carbon atom (see Fig. 10-2). As such, these drugs may have a left- (S) or right- (R) handed configuration. Mepivacaine and bupivacaine are available for clinical use as racemic mixtures (50:50 mixture) of the enantiomers. The enantiomers of a chiral drug may vary in their pharmacokinetics, pharmacodynamics, and toxicity.1 These differences in pharmacologic activity reflect the fact that individual enantiomers bind to receptors or enzymes that are chiral amino acids with stereoselective properties. The S enantiomers of bupivacaine and mepivacaine appear to be less toxic than the commercially available racemic mixtures of these local anesthetics.2 In contrast to mepivacaine and bupivacaine, ropivacaine and levobupivacaine have been developed as a pure S enantiomers.3 These S enantiomers are considered to produce less neurotoxicity and cardiotoxicity than racemic mixtures or the R enantiomers of local anesthetics, perhaps reflecting decreased potency at sodium ion channel.4
Liposomal Local Anesthetics
Various formulation and drug delivery system including liposomes, cyclodextrins, and biopolymers are studied to prolong the duration and to limit the toxicity of local anesthetics. The goal is to upload higher amount of local anesthetic into the molecule and to have a consistent release of local anesthetic in the tissues.5 Liposomes, hydrophobic-based polymer particles such as Poly(lactic-co-glycolic acid) microspheres and solid polymers such as Poly(sebacic-co-ricinoleic acid) P(SA:RA) and their combination with synthetic and natural local anesthetic are examples of delivery systems currently in development or in clinical use.6
Drugs such as lidocaine, tetracaine, and bupivacaine have been incorporated into liposomes to prolong the duration of action and decrease toxicity.7 Bupivacaine extended release liposome injection was recently approved by U.S. Food and Drug Administration (FDA) for local infiltration anesthesia for hemorrhoidectomy and bunionectomy.8–10 Bupivacaine extended release liposome injection consists of microscopic, spherical, and multivesicular liposomes and each liposome particle is composed of a honeycomb-like structure of numerous internal aqueous chambers. Lipid membranes separate these aqueous chambers, and the chambers contain encapsulated bupivacaine. Bupivacaine is released from the liposome particles by a complex mechanism over an extended period (up to 96 hours). In a randomized, double-blind, placebo-controlled, parallel-group study, bupivacaine extended release liposome injection demonstrated a statistically significant reduction in pain through 72 hours, decreased opioid requirements, delayed time to first opioid use, and improved patient satisfaction compared with placebo after hemorrhoidectomy10 (Fig. 10-3). Bupivacaine extended release liposome injection has also been studied for use in nerve blocks,11 intraoperative administration in ileostomy reversal,12 but the results are inconclusive due to smaller sample size. At the time of this writing, bupivacaine extended release liposome injection has limited use, until more conclusive evidence is available for its clinical use.

Mechanism of Action
Local anesthetics bind to specific sites in voltage-gated Na+ channels. They block Na+ current, thereby reducing excitability of neuronal, cardiac or central nervous system tissue.13 Local anesthetics prevent transmission of nerve impulses (conduction blockade) by inhibiting passage of sodium ions through ion-selective sodium channels in nerve membranes.14 The sodium channel itself is a specific receptor for local anesthetic molecules. Failure of sodium ion channel permeability to increase slows the rate of depolarization such that threshold potential is not reached and thus an action potential is not propagated (Fig. 10-4). Local anesthetics do not alter the resting transmembrane potential or threshold potential.

Sodium Channels
The sodium channel is a dynamic transmembrane protein consisting of the large sodium-conducting pore (α subunit) and varying numbers of adjacent smaller β subunits. Nine distinct functional subtypes of voltage-gated Na+ channels are recognized, corresponding to nine genes for their pore-forming α subunits. These have different tissue distributions in the adult and are differentially regulated at the cellular level by receptor-coupled cell signaling systems.15 Different isoforms of voltage-gated Na+ channels, based on biophysical and pharmacologic studies, can provide distinct targets for interventions in various pain syndromes.16 The large polypeptide that forms the α subunit is further divided into four subunits (DI to DIV) (Fig. 10-5). H is the α subunit that allows ion conduction and binds to local anesthetics. However, β subunits may modulate local anesthetic binding to the α subunit. Binding affinities of local anesthetics to the sodium ion channels are stereospecific and depend on the conformational state of the sodium channel.17 Sodium channels exist in activated-open, inactivated-closed, and rested-closed states during various phases of the action potential.18 Voltage-gated Na+ channels undergo fast and slow inactivation processes and this is critical for membrane excitability. The structural changes associated with the inactivation process are poorly understood.19 In the resting nerve membrane, sodium channels are distributed in equilibrium between the rested-closed and inactivated-closed states. By selectively binding to sodium channels in inactivated-closed states, local anesthetic molecules stabilize these channels in this configuration and prevent their change to the rested-closed and activated-open states in response to nerve impulses. Sodium channels in the inactivated-closed state are not permeable to sodium, and thus conduction of nerve impulses in the form of propagated action potentials cannot occur. It is speculated that local anesthetics bind to specific sites located on the inner portion of sodium channels (internal gate or H gate) as well as obstructing sodium channels near their external openings to maintain these channels in inactivated-closed states.14 This binding appears to be weak and to reflect a relatively poor fit of the local anesthetic molecule with the receptor. This is consistent with the broad variety of chemical structures that exhibit local anesthetic activity on sodium channels.17

Frequency-Dependent Blockade
Sodium ion channels tend to recover from local anesthetic–induced conduction blockade between action potentials. Additional conduction blockade is developed each time sodium channels open during an action potential (frequency-dependent blockade). Local anesthetic molecules can gain access to receptors only when sodium channels are in activated-open states and local anesthetic binds more strongly to inactivated state. For this reason, selective conduction blockade of nerve fibers by local anesthetics may be related to the nerve’s characteristic frequencies of activity as well as to its anatomic properties such as diameter. Indeed, a resting nerve is less sensitive to local anesthetic-induced conduction blockade than is a nerve that has been repetitively stimulated. The pharmacologic effects of other drugs, including anticonvulsants and barbiturates in addition to local anesthetics, may reflect frequency-dependent blockade.
Other Site of Action Targets
In addition to sodium ion channels, local anesthetics block voltage-dependent potassium ion channels. Compared with sodium ion channels, local anesthetics exhibit a much lower affinity for potassium channels. However, blockade of potassium ion channels might explain broadening of the action potential in the presence of local anesthetics. Considering the structural similarity between voltage-dependent calcium ion channels and sodium ion channels, it is not surprising that calcium ion currents (L-type is most sensitive) may also be blocked by local anesthetics.20 Although local anesthetics are considered principally ion channel blockers, there is evidence that these drugs may also act on G protein–coupled receptors.21
Minimum Effective Concentration
The minimum concentration of local anesthetic necessary to produce conduction blockade of nerve impulses is termed the Cm. The Cm is analogous to the minimum alveolar concentration (MAC) for inhaled anesthetics. Nerve fiber diameter influences Cm, with larger nerve fibers requiring higher concentrations of local anesthetic for production of conduction blockade. An increased tissue pH or high frequency of nerve stimulation decreases Cm.
Each local anesthetic has a unique Cm, reflecting differing potencies of each drug. The Cm of motor fibers is approximately twice that of sensory fibers; thus, sensory anesthesia may not always be accompanied by skeletal muscle paralysis. Despite an unchanged Cm, less local anesthetic is needed for subarachnoid anesthesia than for epidural anesthesia, reflecting greater access of local anesthetics to unprotected nerves in the subarachnoid space.
Peripheral nerves are composed of myelinated A and B fibers and unmyelinated C fibers. A minimal length of myelinated nerve fiber must be exposed to an adequate concentration of local anesthetic for conduction blockade of nerve impulses to occur. For example, if only one node of Ranvier is blocked (site of change in sodium permeability), the nerve impulse can jump (skip) across this node and conduction blockade does not occur. For conduction blockade to occur in an A fiber, it is necessary to expose at least two and preferably three successive nodes of Ranvier (approximately 1 cm) to an adequate concentration of local anesthetic. Both types of pain-conducting fibers (myelinated A-δ and unmyelinated C fibers) are blocked by similar concentrations of local anesthetics, despite the differences in the diameters of these fibers. Preganglionic B fibers are more readily blocked by local anesthetics than any fiber, even though these fibers are myelinated.
Differential Conduction Blockade
Differential conduction blockade is illustrated by selective blockade of preganglionic sympathetic nervous system B fibers using low concentrations of local anesthetics. Slightly higher concentrations of local anesthetics interrupt conduction in small C fibers and small- and medium-sized A fibers, with loss of sensation for pain and temperature. Nevertheless, touch, proprioception, and motor function are still present such that the patient will sense pressure but not pain with surgical stimulation. In an anxious patient, however, any sensation may be misinterpreted as failure of the local anesthetic.
Changes during Pregnancy
Increased sensitivity (more rapid onset of conduction blockade) may be present during pregnancy.22 Alterations in protein-binding characteristics of bupivacaine may result in increased concentrations of pharmacologically active unbound drug in the parturient’s plasma.23 Nevertheless, progesterone, which binds to the same α1-acid glycoprotein as bupivacaine, does not influence protein binding of this local anesthetic.23 This evidence suggests that bupivacaine and progesterone bind to discrete but separate sites on protein molecules.
Pharmacokinetics
Local anesthetics are weak bases that have pK values somewhat above physiologic pH (see Table 10-1). As a result, <50% of the local anesthetic exists in a lipid-soluble nonionized form at physiologic pH. For example, at pH 7.4, only 5% of tetracaine exists in a nonionized form. Acidosis in the environment into which the local anesthetic is injected (as is present with tissue infection) further increases the ionized fraction of drug. This is consistent with the poor quality of local anesthesia that often results when a local anesthetic is injected into an acidic infected area. Local anesthetics with pKs nearest to physiologic pH have the most rapid onset of action, reflecting the presence of an optimal ratio of ionized to nonionized drug fraction (see Table 10-1).
Intrinsic vasodilator activity will also influence apparent potency and duration of action. For example, the enhanced vasodilator action of lidocaine compared with mepivacaine results in the greater systemic absorption and shorter duration of action of lidocaine. Bupivacaine and etidocaine produce similar vasodilation, but plasma concentrations of bupivacaine after epidural placement exceed those of etidocaine. Presumably, the greater lipid solubility of etidocaine results in tissue sequestration and less available drug for systemic absorption. Occasional prolonged sensory blockade after injection of etidocaine has been attributed to tissue sequestration.
Absorption and Distribution
Absorption of a local anesthetic from its site of injection into the systemic circulation is influenced by the site of injection and dosage, use of epinephrine, and pharmacologic characteristics of the drug (Fig. 10-6). The ultimate plasma concentration of a local anesthetic is determined by the rate of tissue distribution and the rate of clearance of the drug. For example, the infusion of lidocaine for 1 minute is followed by a rapid decrease in the drug’s plasma concentration that is paralleled by an initial high uptake into the lungs and distribution of the local anesthetic to highly perfused tissues (brain, heart, and kidneys).24 Lipid solubility of the local anesthetic is important in this redistribution, as well as being a primary determinant of intrinsic local anesthetic potency. After distribution to highly perfused tissues, the local anesthetic is redistributed to less well perfused tissues, including skeletal muscles and fat. Consideration of cardiac output is important for describing the overall tissue distribution of local anesthetics and presumably their intercompartmental clearance.25 Ultimately, the local anesthetic is eliminated from the plasma by metabolism and excretion.

In addition to the tissue blood flow and lipid solubility of the local anesthetic, patient-related factors such as age, cardiovascular status, and hepatic function will also influence the absorption and resultant plasma concentrations of local anesthetics. Protein binding of local anesthetics will influence their distribution and excretion. In this regard, protein binding parallels lipid solubility of the local anesthetic and is inversely related to the plasma concentration of drug (see Table 10-1) (Fig. 10-7).26 Overall, after systemic absorption, amide local anesthetics are more widely distributed in tissues than ester local anesthetics.

Lung Extraction
The lungs are capable of extracting local anesthetics such as lidocaine, bupivacaine, and prilocaine from the circulation.27 After rapid entry of local anesthetics into the venous circulation, this pulmonary extraction will limit the concentration of drug that reaches the systemic circulation for distribution to the coronary and cerebral circulations. For bupivacaine, this first-pass pulmonary extraction is dose dependent, suggesting that the uptake process becomes saturated rapidly.28 Propranolol impairs bupivacaine extraction by the lungs, perhaps reflecting a common receptor site for the two drugs.29 Furthermore, propranolol decreases plasma clearance of lidocaine and bupivacaine, presumably reflecting propranolol-induced decreases in hepatic blood flow or inhibition of hepatic metabolism.30
Placental Transfer
There may be clinically significant transplacental transfer of local anesthetics between the mother and fetus. Plasma protein binding influences the rate and degree of diffusion of local anesthetics across the placenta (see Table 10-1). Bupivacaine, which is highly protein bound (approximately 95%), has an umbilical vein–maternal arterial concentration ratio of about 0.32 compared with a ratio of 0.73 for lidocaine (approximately 70% protein bound) and a ratio of 0.85 for prilocaine (approximately 55% protein bound).31 Ester local anesthetics, because of their rapid hydrolysis, are not available to cross the placenta in significant amounts. Acidosis in the fetus, which may occur during prolonged labor, can result in accumulation of local anesthetic molecules in the fetus (ion trapping) (Fig. 10-8).32

Renal Elimination and Clearance
The poor water solubility of local anesthetics usually limits renal excretion of unchanged drug to less than 5%.33 The exception is cocaine, of which 10% to 12% of unchanged drug can be recovered in urine. Water-soluble metabolites of local anesthetics, such as paraaminobenzoic acid resulting from metabolism of ester local anesthetics, are readily excreted in urine. Clearance values and elimination half-times for amide local anesthetics probably represent mainly hepatic metabolism, because renal excretion of unchanged drug is minimal (see Table 10-1). Pharmacokinetic studies of ester local anesthetics are limited because of a short elimination half-time due to their rapid hydrolysis in the plasma and liver.
Metabolism of Amide Local Anesthetics
Amide local anesthetics undergo varying rates of metabolism by microsomal enzymes located primarily in the liver. Prilocaine undergoes the most rapid metabolism; lidocaine and mepivacaine are intermediate; and etidocaine, bupivacaine, and ropivacaine undergo the slowest metabolism among the amide local anesthetics. The initial step is conversion of the amide base to aminocarboxylic acid and a cyclic aniline derivative. Complete metabolism usually involves additional steps, such as hydroxylation of the aniline moiety and N-dealkylation of the aminocarboxylic acid.
Compared with that of ester local anesthetics, the metabolism of amide local anesthetics is more complex and slower. This slower metabolism means that sustained increases of the plasma concentrations of amide local anesthetics, and thus systemic toxicity, are more likely than with ester local anesthetics. Furthermore, cumulative drug effects of amide local anesthetics are more likely than with ester local anesthetics.
Lidocaine
The principal metabolic pathway of lidocaine is oxidative dealkylation in the liver to monoethylglycinexylidide followed by hydrolysis of this metabolite to xylidide. Monoethylglycinexylidide has approximately 80% of the activity of lidocaine for protecting against cardiac dysrhythmias in an animal model. This metabolite has a prolonged elimination half-time, accounting for its efficacy in controlling cardiac dysrhythmias after the infusion of lidocaine is discontinued. Xylidide has only approximately 10% of the cardiac antidysrhythmic activity of lidocaine. In humans, approximately 75% of xylidide is excreted in the urine as 4-hydroxy-2,6-dimethylaniline.
Hepatic disease or decreases in hepatic blood flow, which may occur during anesthesia, can decrease the rate of metabolism of lidocaine. For example, the elimination half-time of lidocaine is increased more than fivefold in patients with liver dysfunction compared with normal patients. Decreased hepatic metabolism of lidocaine should be anticipated when patients are anesthetized with volatile anesthetics. Maternal clearance of lidocaine is prolonged in the presence of pregnancy-induced hypertension, and repeated administration of lidocaine can result in higher plasma concentrations than in normotensive parturients.34
Prilocaine
Prilocaine is an amide local anesthetic that is metabolized to orthotoluidine. Orthotoluidine is an oxidizing compound capable of converting hemoglobin to its oxidized form, methemoglobin, resulting in a potentially life-threatening complication, methemoglobinemia (see the section “Methemoglobinemia”). When the dose of prilocaine is >600 mg, there may be sufficient methemoglobin present (3 to 5 g/dL) to cause the patient to appear cyanotic, and oxygen-carrying capacity is decreased. Methemoglobinemia is readily reversed by the administration of methylene blue, 1 to 2 mg/kg intravenously, over 5 minutes (total dose should not exceed 7 to 8 mg/kg). The ability of prilocaine to cause dose-related methemoglobinemia limits its clinical usefulness, with the exception of intravenous (IV) regional anesthesia. Prilocaine causes less vasodilation than other local anesthetics and thus can be utilized without epinephrine added to the local anesthetic solution.
Mepivacaine
Mepivacaine has pharmacologic properties similar to those of lidocaine, although the duration of action of mepivacaine is somewhat longer. Clearance of mepivacaine is decreased in neonates, leading to a prolonged elimination half-time. In contrast to lidocaine, mepivacaine lacks vasodilator activity. As such, mepivacaine is an alternate selection when addition of epinephrine to the local anesthetic solution is not recommended.
Bupivacaine
Possible pathways for metabolism of bupivacaine include aromatic hydroxylation, N-dealkylation, amide hydrolysis, and conjugation.35 Only the N-dealkylated metabolite N-desbutylbupivacaine, has been measured in blood or urine after epidural or spinal anesthesia. The mean total urinary excretion of bupivacaine and its dealkylation and hydroxylation metabolites account for >40% of the total anesthetic dose.35 α1-Acid glycoprotein is the most important plasma protein-binding site of bupivacaine, and its concentration is increased in many clinical situations, including postoperative trauma.36
Ropivacaine
Ropivacaine is metabolized to 2,6-pipecoloxylidide and 3-hydroxyropivacaine by hepatic cytochrome P450 enzymes. Both metabolites have significantly less local anesthetic potency than ropivacaine. Because only a very small fraction of ropivacaine is excreted unchanged in the urine (about 1%) when the liver is functioning normally, dosage adjustments based on renal function do not seem necessary. However, in uremic patients, 2,6-pipecoloxylidide may accumulate and produce toxic effects.37 Overall, clearance of ropivacaine is higher than that determined for bupivacaine, and its elimination half-time is shorter.3 The higher clearance of ropivacaine may offer an advantage over bupivacaine in terms of systemic toxicity. The lipid solubility of ropivacaine is intermediate between lidocaine and bupivacaine. Ropivacaine is highly bound to α1-acid glycoprotein.
Dibucaine
Dibucaine is a quinoline derivative with an amide bond in the connecting hydrocarbon chain. This local anesthetic is metabolized in the liver and is the most slowly eliminated of all the amide derivatives. Dibucaine is better known for its ability to inhibit the activity of normal butyrylcholinesterase (plasma cholinesterase) by more than 70%, compared with only approximately 20% inhibition of the activity of atypical enzyme. Atypical plasma cholinesterases account for prolonged effects and toxicity of drugs such as succinylcholine and chloroprocaine that are metabolized by this enzyme. Laboratory evaluation of patients suspected of having atypical pseudocholinesterase is facilitated by measurement of the degree of enzyme suppression by dibucaine, a test termed the dibucaine number. See further discussion of dibucaine in Chapter 12, Neuromuscular Blocking Drugs and Reversal Agents.
Metabolism of Ester Local Anesthetics
Ester local anesthetics undergo hydrolysis by cholinesterase enzyme, principally in the plasma and to a lesser extent in the liver. The rate of hydrolysis varies, with chloroprocaine being most rapid, procaine being intermediate, and tetracaine being the slowest. The resulting metabolites are pharmacologically inactive, although paraaminobenzoic acid may be an antigen responsible for subsequent allergic reactions. The exception to hydrolysis of ester local anesthetics in the plasma is cocaine, which undergoes significant metabolism in the liver.
Systemic toxicity is inversely proportional to the rate of hydrolysis; thus, tetracaine is more likely than chloroprocaine to result in excessive plasma concentrations. Because cerebrospinal fluid contains little to no cholinesterase enzyme, anesthesia produced by subarachnoid placement of tetracaine will persist until the drug has been absorbed into the systemic circulation. Plasma cholinesterase activity and the hydrolysis rate of ester local anesthetics are slowed in the presence of liver disease or an increased blood urea nitrogen concentration. Plasma cholinesterase activity may be decreased in parturients and in patients being treated with certain chemotherapeutic drugs. Patients with atypical plasma cholinesterase may be at increased risk for developing excess systemic concentrations of an ester local anesthetic due to absent or limited plasma hydrolysis.
Procaine
Procaine is hydrolyzed to paraaminobenzoic acid, which is excreted unchanged in urine, and to diethylaminoethanol, which is further metabolized because only 30% is recovered in urine. Overall, <50% of procaine is excreted unchanged in urine. Increased plasma concentrations of paraaminobenzoic acid do not produce symptoms of systemic toxicity.
Chloroprocaine
Addition of a chlorine atom to the benzene ring of procaine to form chloroprocaine increases by 3.5 times the rate of hydrolysis of the local anesthetic by plasma cholinesterase, as compared with procaine. Resulting pharmacologically inactive metabolites of chloroprocaine are 2-chloro-aminobenzoic acid and 2-diethylaminoethanol. Maternal and neonatal plasma cholinesterase activity may be decreased up to 40% at term, but minimal placental passage of chloroprocaine confirms that even this decreased activity is adequate to hydrolyze most of the chloroprocaine that is absorbed from the maternal epidural space.38,39
Tetracaine
Tetracaine undergoes hydrolysis by plasma cholinesterase, but the rate is slower than for procaine.
Benzocaine
Benzocaine (ethyl aminobenzoate) is unique among clinically useful local anesthetics because it is a weak acid (pKa 3.5), so that it exists predominantly in the nonionized form at physiologic pH. As such, benzocaine is ideally suited for topical anesthesia of mucous membranes prior to tracheal intubation, endoscopy, transesophageal echocardiography, and bronchoscopy. Onset of topical anesthesia is rapid and lasts 30 to 60 minutes. A brief spray of 20% benzocaine delivers the recommended dose of 200 to 300 mg. Systemic absorption of topical benzocaine is enhanced by defects in the skin and mucosa as well as from the gastrointestinal tract should any of the local anesthetic be swallowed. The product Cetacaine is marketed as a combination of 14% benzocaine, 2% tetracaine, and 2% butamben in a topical applicator that acts as an atomizer. Methemoglobinemia is a rare but potentially life-threatening complication following topical application of benzocaine, especially when the dose exceeds 200 to 300 mg (see the section “Methemoglobinemia”).
Cocaine
Cocaine is metabolized by plasma and liver cholinesterases to water-soluble metabolites that are excreted in urine. Plasma cholinesterase activity is decreased in parturients, neonates, the elderly, and patients with severe underlying hepatic disease. Cocaine may be present in urine for 24 to 36 hours, depending on the route of administration and cholinesterase activity. Assays for the metabolites of cocaine in urine are useful markers of cocaine use or absorption (see the section “Cocaine Toxicity”).
Alkalinization of Local Anesthetic Solutions
Alkalinization of local anesthetic solutions shortens the onset of neural blockade, enhances the depth of sensory and motor blockade, and increases the spread of epidural blockade.40 The pH of commercial preparations of local anesthetic solutions ranges from 3.9 to 6.5 and is especially acidic if prepackaged with epinephrine (increased acidity prolongs the shelf life of epinephrine). The pKa of local anesthetics used clinically is near 8, so that only a small fraction (about 3%) of the local anesthetic exists in the lipid-soluble form. Alkalinization increases the percentage of local anesthetic existing in the lipid-soluble form that is available to diffuse across lipid cellular barriers. Adding sodium bicarbonate will speed the onset of peripheral nerve block and epidural block by 3 to 5 minutes.
Adjuvant Mixed with Local Anesthetics
Dexmedetomidine has been used as an adjuvant in local anesthetic admixtures and a central effect is postulated for prolongation of the local anesthetic affect. IV dexmedetomidine, in a recent systematic review and meta-analysis, showed increased duration of motor and sensory block and also increased duration for first analgesic request after spinal anesthesia.41 Magnesium has also shown promising initial results when introduced in to the intrathecal space as an addition to local anesthetic with or without opioids. Duration of spinal anesthesia was increased in magnesium group.42 In pediatric patients, addition of clonidine and ketamine to the regional anesthesia showed good pharmacokinetic and pharmacodynamic profiles of efficacy and safety, improving and prolonging the action of associated local anesthetics.43
Combinations of Local Anesthetics
Local anesthetics may be combined in an effort to produce a rapid onset (chloroprocaine) and prolonged duration (bupivacaine) of action. Nevertheless, placement of chloroprocaine in the epidural space may decrease the efficacy of subsequent epidural bupivacaine-induced analgesia during labor. It is speculated that the low pH of the chloroprocaine solution could decrease the nonionized pharmacologically active fraction of bupivacaine. Tachyphylaxis to the local anesthetic mixture could also reflect local acidosis due to the low pH of the bathing solution. For these reasons, adjustment of the pH of the chloroprocaine solution with the addition of 1 mL of 8.4% sodium bicarbonate added to 30 mL of chloroprocaine solution just before placement into the epidural space may improve the efficacy of the chloroprocaine-bupivacaine combination.44 Local anesthetic toxicity of combinations of drugs are additive rather than synergistic.45
Use of Vasoconstrictors
The duration of action of a local anesthetic is proportional to the time the drug is in contact with nerve fibers. For this reason, epinephrine (1:200,000 or 5 µg/mL) may be added to local anesthetic solutions to produce vasoconstriction, which limits systemic absorption and maintains the drug concentration in the vicinity of the nerve fibers to be anesthetized. Indeed, addition of epinephrine to a lidocaine or mepivacaine solution prolongs the duration of conduction blockade and decreases systemic absorption of local anesthetics by 20% to 30% (Fig. 10-9).46,47 For bupivacaine, addition of epinephrine also increases the duration of conduction blockade but to a lesser degree and the reduction in systemic absorption is by 10% to 20%. Most local anesthetics, with the exception of ropivacaine, possess intrinsic vasodilator properties, and it is possible that epinephrine-induced vasoconstriction will slow clearance from the injection site, thus prolonging the time the drug is in contact with nerve fibers.

The impact of adding epinephrine to the local anesthetic solution is influenced by the specific local anesthetic selected and the level of sensory blockade required if a spinal or epidural anesthetic is chosen. For example, the impact of epinephrine in prolonging the duration of conduction blockade and decreasing systemic absorption of bupivacaine and etidocaine is less than that observed with lidocaine, presumably because the greater lipid solubility of bupivacaine and etidocaine causes them to bind avidly to tissues. The duration of sensory anesthesia in the lower extremities, but not the abdominal region, is extended when epinephrine (0.2 mg) or phenylephrine (2 mg) is added to local anesthetic solutions of bupivacaine or lidocaine placed into the subarachnoid space. Vasoconstrictors prolong the effect of tetracaine for spinal anesthesia. Epinephrine added to a low dose of tetracaine (6 mg) increases the success rate of spinal anesthesia, whereas the success rate is not altered by epinephrine when the subarachnoid dose of tetracaine is 10 mg.48 In addition to decreasing systemic absorption to prolong conduction blockade, epinephrine may also enhance conduction blockade by increasing neuronal uptake of the local anesthetic. The α-adrenergic effects of epinephrine may be associated with some degree of analgesia that could contribute to the effects of the conduction blockade. The addition of epinephrine to local anesthetic solutions has little, if any, effect on the onset rate of local anesthesia.
Decreased systemic absorption of local anesthetic due to vasoconstriction produced by epinephrine increases the likelihood that the rate of metabolism will match that of absorption, thus decreasing the possibility of systemic toxicity. Whenever local anesthetic solutions containing epinephrine are administered in the presence of inhaled anesthetics, the possibility of enhanced cardiac irritability should be considered. Systemic absorption of epinephrine may accentuate systemic hypertension in vulnerable patients.
Adverse Effects of Local Anesthetics
The principal side effects related to the use of local anesthetics are allergic reactions and systemic toxicity due to excessive plasma and tissue concentrations of the local anesthetic. Systemic toxicity in association with regional anesthesia is estimated to result in seizures in 1 to 4 per 1,000 patient exposures to local anesthetics, with bupivacaine being the drug most likely to be associated with this adverse response.49
Allergic Reactions
Allergic reactions to local anesthetics are rare despite the frequent use of these drugs. It is estimated that less than 1% of all adverse reactions to local anesthetics are due to an allergic mechanism.50 Instead, the overwhelming majority of adverse responses that are often attributed to an allergic reaction are instead manifestations of excess plasma concentrations of the local anesthetic.
Esters of local anesthetics that produce metabolites related to paraaminobenzoic acid are more likely than amide local anesthetics, which are not metabolized to paraaminobenzoic acid, to evoke an allergic reaction. An allergic reaction after the use of a local anesthetic may be due to methylparaben or similar substances used as preservatives in commercial preparations of ester and amide local anesthetics. These preservatives are structurally similar to paraaminobenzoic acid. As a result, an allergic reaction may reflect prior stimulation of antibody production by the preservative and not a reaction to the local anesthetic.
Cross-Sensitivity
Cross-sensitivity between local anesthetics reflects the common metabolite paraaminobenzoic acid. A similar cross-sensitivity, however, does not exist between classes of local anesthetics. Therefore, a patient with a known allergy to an ester local anesthetic can receive an amide local anesthetic without an increased risk of an allergic reaction. Likewise, an ester local anesthetic can be administered to a patient with a known allergy to an amide local anesthetic. It is important that the “safe” local anesthetic be preservative-free.
Documentation of Allergy
Documentation of allergy to a local anesthetic is based on the clinical history and perhaps the use of intradermal testing. The occurrence of rash, urticaria, and laryngeal edema, with or without hypotension and bronchospasm, is highly suggestive of a local anesthetic–induced allergic reaction. Conversely, hypotension associated with syncope or tachycardia when an epinephrine-containing local anesthetic solution is administered suggests an accidental intravascular injection of drug. Use of an intradermal test requires injection of preservative-free preparations of local anesthetic solutions to eliminate the possibility that the allergic reaction was caused by a substance other than the local anesthetic.
Local Anesthetic Systemic Toxicity
Local anesthetic systemic toxicity (LAST) is due to an excess plasma concentration of the drug. Plasma concentrations of local anesthetics are determined by the rate of drug entrance into the systemic circulation relative to their redistribution to inactive tissue sites and clearance by metabolism. Accidental direct intravascular injection of local anesthetic solutions during performance of peripheral nerve block anesthesia or epidural anesthesia is the most common mechanism for production of excess plasma concentrations of local anesthetics. A variety of factors influence the likelihood and severity of LAST, including individual patient risk factors, concurrent medications, location and technique of block, specific local anesthetic compound, total local anesthetic dose (the product of concentration and volume), timeliness of detection, and adequacy of treatment.51 The magnitude of this systemic absorption depends on the (a) dose administered into the tissues, (b) vascularity of the injection site, (c) presence of epinephrine in the solution, and (d) physicochemical properties of the drug (see Table 10-1). For example, systemic absorption of local anesthetics is greatest after injection for an intercostal nerve bock, intermediate for epidural anesthesia, and least for a brachial plexus block.52 Addition of 5 µg of epinephrine to every milliliter of local anesthetic solution (1:200,000 dilution) decreases systemic absorption of local anesthetics by approximately one-third47 (see the section “Use of Vasoconstrictors”). Systemic toxicity of local anesthetics involves the central nervous system (CNS) and cardiovascular system.
Local anesthetics differ with regard to their CNS toxicity and cardiac toxicity. The cardiovascular/central nervous system (CV/CNS) ratio describes the dose required to produce CV arrhythmias versus that required to produce seizures.53 If the ratio is lower, it implies a reduced safety margin for the local anesthetic compounds compared to the compound with higher ratio, to detect impending cardiotoxicity based on premonitory CNS signs. Bupivacaine is a more potent local anesthetic and generates arrhythmias at lower concentrations compared with lidocaine and mepivacaine. Also in animal studies (dogs), at comparable dosages, bupivacaine and etidocaine cause severe arrhythmias without decreased myocardial contractility, whereas lidocaine caused the opposite, that is, depressed myocardial contractility without arrhythmia.54
Central Nervous System Effects
Low plasma concentrations of local anesthetics are likely to produce numbness of the tongue and circumoral tissues, presumably reflecting delivery of drug to these highly vascular tissues (Table 10-2). As the plasma concentrations continue to increase, local anesthetics readily cross the blood–brain barrier and produce a predictable pattern of CNS changes. Restlessness, vertigo, tinnitus, and difficulty in focusing occur initially. Further increases in the CNS concentration of local anesthetic result in slurred speech and skeletal muscle twitching. Skeletal muscle twitching is often first evident in the face and extremities and signals the imminence of tonic-clonic seizures. Vivid fear of imminent death and a delusional belief of having died have been described in patients experiencing toxic reactions to local anesthetics administered for regional anesthesia and pain relief.55

Lidocaine and other amide local anesthetics may cause drowsiness before the onset of seizures. Seizures are classically followed by CNS depression, which may be accompanied by hypotension and apnea. The onset of seizures may reflect selective depression of inhibitory cortical neurons by local anesthetics, leaving excitatory pathways unopposed. An alternative explanation for seizures is local anesthetic–induced inhibition of the release of neurotransmitters, particularly γ-aminobutyric acid. The precise site of local anesthetic–induced seizures is not known, although it appears to be in the temporal lobe or the amygdala.
Plasma concentrations of local anesthetics producing signs of central nervous system (CNS) toxicity depend on the specific drug involved. Lidocaine, mepivacaine, and prilocaine demonstrate effects on the CNS at plasma concentrations of 5 to 10 µg/mL. The typical plasma concentration of bupivacaine associated with seizures is 4.5 to 5.5 µg/mL.52 Ropivacaine and bupivacaine produce convulsions in awake animals at similar doses.3 The threshold plasma concentration at which CNS toxicity occurs may be related more to the rate of increase of the serum concentration than to the total amount of drug injected.33
The active metabolites of lidocaine, including monoethylglycinexylidide, may exert an additive effect in causing systemic toxicity after epidural administration of lidocaine. For this reason, it has been recommended that the plasma venous concentration of lidocaine be monitored when the cumulative epidural dose of lidocaine is >900 mg.56 The seizure threshold for lidocaine may be related to CNS levels of serotonin (5-hydroxytryptophan). For example, accumulation of serotonin decreases the seizure threshold of lidocaine and prolongs the duration of seizure activity.
There is an inverse relationship between the PaCO2 and seizure thresholds of local anesthetics, presumably reflecting variations in cerebral blood flow and resultant delivery of drugs to the brain. Increases in the serum potassium concentration can facilitate depolarization and thus markedly increase local anesthetic toxicity. Conversely, hypokalemia, by creating hyperpolarization, can greatly decrease local anesthetic toxicity. The threshold for neurotoxicity of lidocaine may be decreased when patients being treated with the antidysrhythmic drug mexiletine receive lidocaine during the perioperative period.57
Cardiovascular System Effects
The cardiovascular system is more resistant to the toxic effects of high plasma concentrations of local anesthetics than is the CNS. For example, lidocaine in plasma concentrations of <5 µg/mL is devoid of adverse cardiac effects, producing only a decrease in the rate of spontaneous phase 4 depolarization (automaticity). Nevertheless, plasma lidocaine concentrations of 5 to 10 µg/mL, and equivalent plasma concentrations of other local anesthetics, may produce profound hypotension due to relaxation of arteriolar vascular smooth muscle and direct myocardial depression (see Table 10-2). As a result, hypotension reflects both decreased systemic vascular resistance and decreased cardiac output.
Part of the cardiac toxicity that results from high plasma concentrations of local anesthetics occurs because these drugs also block cardiac sodium channels. At low concentrations of local anesthetics, this effect on sodium channels probably contributes to cardiac antidysrhythmic properties of these drugs. However, when the plasma concentrations of local anesthetics are excessive, sufficient cardiac sodium channels become blocked so that conduction and automaticity become adversely depressed. For example, excessive plasma concentrations of lidocaine may slow conduction of cardiac impulses through the heart, manifesting as prolongation of the P-R interval and QRS complex on the electrocardiogram. Effects of local anesthetics on calcium ion and potassium ion channels and local anesthetic-induced inhibition of cyclic adenosine monophosphate (cAMP) production may also contribute to cardiac toxicity.58
Selective Cardiac Toxicity
Accidental IV injection of bupivacaine may result in precipitous hypotension, cardiac dysrhythmias, and atrioventricular heart block.59 After accidental IV injection, the protein-binding sites (α1-acid glycoprotein and albumin) for bupivacaine are quickly saturated, leaving a significant mass of unbound drug available for diffusion into the conducting tissue of the heart. IV injection of bupivacaine or lidocaine to awake animals produces serious cardiac dysrhythmias only in animals receiving bupivacaine. Premature ventricular contractions, widening of the QRS complex, and ventricular tachycardia are the most common arrhythmias seen, though other arrhythmias including supraventricular tachycardia, atrioventricular heart block, and ST-T wave changes can also occur but are less common.60 Cardiotoxic plasma concentrations of bupivacaine are 8 to 10 µg/mL.61
Physiologic changes and concomitant drug therapy may make patients more vulnerable to bupivacaine cardiac toxicity. For example, pregnancy may increase sensitivity to cardiotoxic effects of bupivacaine, but not ropivacaine, as emphasized by occurrence of cardiopulmonary collapse with a smaller dose of bupivacaine in pregnant compared with nonpregnant animals (Fig. 10-10).3,62 The threshold for cardiac toxicity produced by bupivacaine may be decreased in patients being treated with drugs that inhibit myocardial impulse propagation (β-adrenergic blockers, digitalis preparations, calcium channel blockers).63 Indeed, in the presence of propranolol, atrioventricular heart block and cardiac dysrhythmias occurred at plasma bupivacaine concentrations of 2 to 3 µg/mL.61 This suggests that caution must be taken in the use of bupivacaine in patients who are on antidysrhythmic drugs or other cardiac medications known to depress impulse propagation. Epinephrine and phenylephrine may increase bupivacaine cardiotoxicity, reflecting bupivacaine-induced inhibition of catecholamine-stimulated production of cAMP.64 The cardiac toxicity of bupivacaine in animals is enhanced by arterial hypoxemia, acidosis, or hypercarbia.

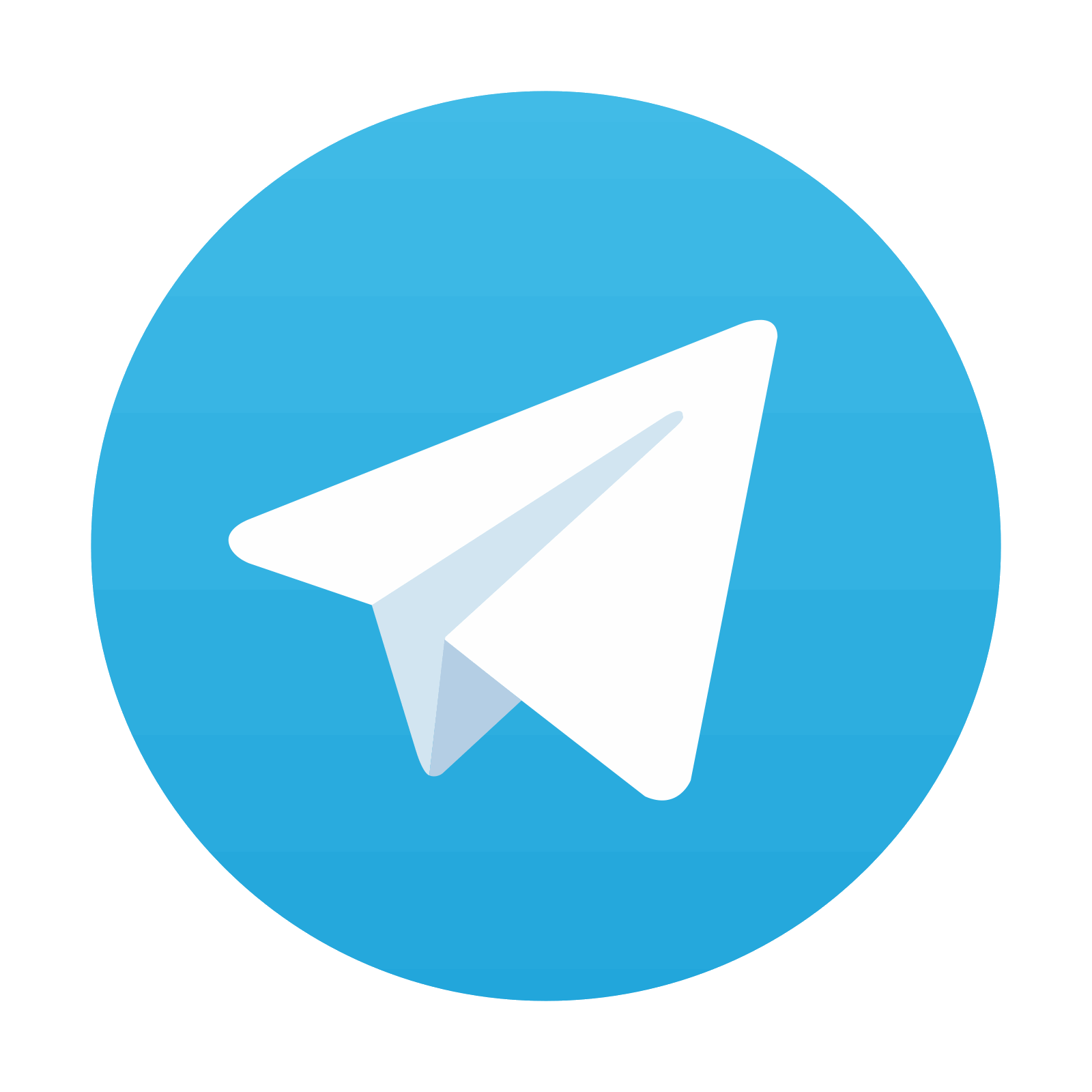
Stay updated, free articles. Join our Telegram channel
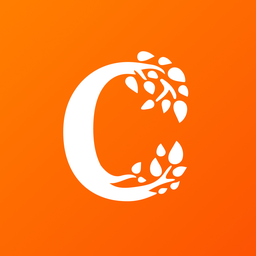
Full access? Get Clinical Tree
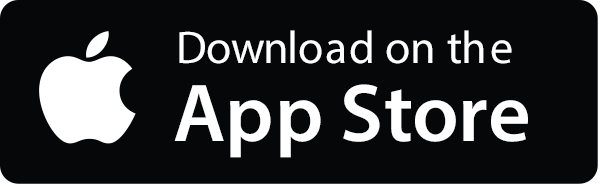
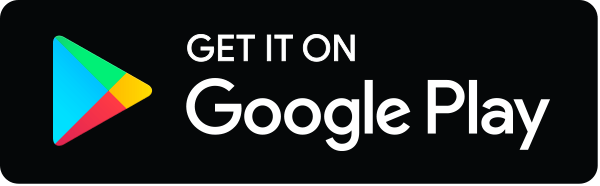