Chapter 20 Laser Risks and Preventive Measures for the Staff
HISTORY OF LASERS
The word LASER is an acronym for Light Amplification by Stimulated Emission of Radiation (Bertolotti, 2004). A laser beam is merely light; however, it is light that is amplified by stimulated emission, unlike normal light, which is produced by spontaneous emission. The differences between laser light and normal light are part of the reason that the laser has so rapidly assumed, and is continuing to assume, such a prominent position in science and medicine. Understanding the physics of the laser is necessary to understanding and optimizing safe practice within the perioperative setting.
The concept of a laser actually began in 1913 when Niels Bohr developed his theory of the atom (Carruth and McKenzie, 1986). This theory uses the model so familiar today of the atom consisting of a nucleus around which electrons circulate. Based on this model, Bohr was able to describe the atomic basis for the production of light energy by spontaneous emission. Using Bohr’s model, in 1917 Albert Einstein speculated on the possibility of both spontaneous and stimulated emission. However, the existence of stimulated emission remained primarily a theory until 1954, when Charles H. Townes, Nikolay Basov, and Alexander Prokhorov were able to physically produce measurable stimulated emission with the MASER (Microwave Amplification by Stimulated Emission of Radiation) (Carruth and McKenzie, 1986).
Based on work with the maser, Charles H. Townes and Arthur L. Schawlow published a paper in 1958 discussing the possibility of extending the maser principles into the optical portion of the electromagnetic spectrum (Bertolotti, 2004). A year earlier, Gordon Gould, a graduate student at Columbia University, developed the principle of laser. He recognized that he had something great, so he had the notebook containing this theory notarized. He received bad legal advice, however, and did not pursue a patent until 9 months after Townes and Schawlow had submitted their patent. After many years of legal battles, Gould finally received credit and recognition for coining the word laser (Ball, 2004). However, it was not until 1960 that Theodore H. Maiman of Hughes Research Laboratories developed the first visible laser, a ruby laser (Maiman, 1960). Following this breakthrough, the entire area of visible and near-visible lasers grew rapidly. The development of other lasers quickly followed: the helium-neon (He-Ne) and neodymium:yttrium-aluminum-garnet (Nd:YAG) lasers were developed in 1961. The first semiconductor laser was developed in 1962, and 1963 brought the development of the carbon dioxide (CO2) laser.
LASER PHYSICS
Light Properties
When light is absorbed by a surface or material, the energy of the light beam is normally transformed into heat energy. This thermal effect of light energy absorption is the principle behind laser surgery. The laser light is absorbed by the tissue and transformed into thermal energy, causing the desired ablation or coagulation of tissue. Thus laser surgery is similar to many other means of delivering heat energy to the tissue. However, it is by far the most efficient and most precise means of delivering thermal energy to date. It is this efficiency and preciseness that makes the laser so valuable to all fields of surgery (Laserscope, 2002).
The transmission of laser light through a surface is a factor that is very much dependent on the frequency or wavelength of the laser light and the properties of the tissue at that frequency. For example, glass transmits light in the visible spectrum almost completely, while completely blocking the transmission of the far-infrared energy produced by the holmium:yttrium-aluminum-garnet (Ho:YAG) or CO2 laser. When light transmits into any solid surface, the light will interact. Each particle will then reflect, absorb, or transmit the light off or through the surface layer. This random reflection, absorption, and transmission scatters the light in all directions within the material. The deeper the light is able to transmit within the material, the more the scattering and spreading of the light energy. Laser energy at wavelengths that transmit deeply into biological tissue will cause thermal destruction at greater depths, but will also cause a subsurface area of thermal damage, the radius of which is larger than the radius of the laser beam spot size (Laserscope, 2002).
Laser Energy Generation
The name of a particular laser is derived from the active medium within the laser tube, which is responsible for the actual lasing. The medium can be a solid, a liquid, or a gas. A solid medium is usually in crystal form, although semiconductor lasers, so important in the communication industry, are also considered solid-state lasers. The potassium-titanyl-phosphate (KTP) laser and the Nd:YAG lasers are among the most common solid-state lasers. Liquid lasers are almost exclusively dye lasers. Dye lasers are very versatile, because, by changing the concentration or type of dye within the resonant chamber, the laser can actually be tuned to almost any desired frequency. However, a major drawback to dye lasers is a severe limitation in the amount of output power possible. Dye lasers are often used in ophthalmology and dermatology procedures. Gas lasers are by far the most common and popular types of lasers. Examples of gas lasers include the CO2, the helium-neon, and the argon laser. Gas lasers are generally considered to be the most economical, efficient, and reliable type of laser (Carruth and McKenzie, 1986).
Characteristics of Laser Light
Laser light produced by stimulated emission possesses three properties that distinguish it from ordinary light produced by spontaneous emission. These three properties are monochromaticity, coherence, and collimation (Laserscope, 2002).
Monochromaticity means that all of the photons of light emitted by the laser are at one frequency and are moving in the same space and time. However, laser light is as close to being at one frequency as is physically possible. White light produced by spontaneous emission consists of light of all visible frequencies or all colors mixed together. Even visible light that might be considered a single color actually consists of a considerably wider spectrum of frequencies than light produced by stimulated emission. Therefore laser light is one color of light—monochromatic (Laserscope, 2002).
Coherence is a term referring to the wave nature of light and describes the fact that all of the peaks of the sine waves representing each photon are exactly in phase with each other, or both in the same space and time. In other words, all of the peaks of the waves exactly coincide, allowing all of the numerous small oscillations to accumulate. White light produced by spontaneous emission consists of all different frequencies. Therefore it is impossible for the waves of the white light to sum up consistently as does monochromatic light, because each frequency has its own wavelength, and the difference in wavelength will ensure that the photons of different frequencies will be out of phase the majority of the time. White light is composed of many colors of the light spectrum and will cancel each frequency out. With laser light, because of the coherency, the frequency will become in sync. This accounts for the brilliance so often seen with a laser beam (Laserscope, 2002).
Collimation describes the fact that all of the photons, or waves, produced by the stimulated emission process are going in exactly the same direction, parallel to each other. This property allows the stimulated emission photons to remain in phase while traveling for a long distance away from the laser source. Light produced by spontaneous emission tends to spread in all directions, as does the light produced by a light bulb. Although spontaneous emission light can be “collimated” using special optical lenses or mirrors to form a beam, it can never be as well collimated as light produced by stimulated emission (Laserscope, 2002).
Several characteristics of laser light result from these three properties. Although white light can be used to form images, laser light cannot form images, primarily because the laser light is collimated and coherent. Image formation requires light to travel in a wide range of directions from a given point. Because laser light photons all travel in the same direction, the light cannot be used to form an image. It can, however, be used to form holograms, but these are not “true” images (Bertolotti, 2004).
Operating Parameters
Spot Size
Spot size is the second operating parameter and describes the diameter of the minimum spot size achievable with a given lens. This parameter is typically given in millimeters. It is important to remember that spot size specifications are usually given for the spot size diameter, not the spot size radius, which is typically used in power density calculations. The spot size determines how concentrated the beam’s power will be at the focal point of the laser beam. The minimum spot size is usually fixed by the focal length of the focusing lens. The spot size can be changed by using another lens with a different focal point (Laserscope, 2002).
Power Density
Power density is a parameter that combines two of the three operating parameters to describe how concentrated the laser beam is on the surface of the tissue. Power density determines the effect of the laser beam when applied to the tissue. High power densities of the CO2 laser correlate with vaporization of tissue, whereas lower power densities are associated with tissue coagulation. Power density is expressed in watts per square centimeter (W/cm2), although occasionally this parameter is given in watts per square millimeter (Ball, 2004).
Increasing the power density will increase the laser beam’s ability to vaporize as opposed to coagulate. The power density is directly proportional to the power setting. Therefore doubling the power will double the power density. However, the power density is inversely proportional to the square of the spot size diameter. Thus decreasing the spot size by half will quadruple the power density (Bertolotti, 2004).
Radiant exposure is directly proportional to the amount of thermal damage done to the tissue and is a better description of the tissue effect than is power density (Bertolotti, 2004). The actual time the laser impacts tissue is extremely difficult to estimate, particularly when the laser beam is in almost constant motion over the tissue surface as it is during most surgical procedures. Most descriptions of laser surgical procedures deal with the parameter of power density, leaving variations in the application of the time parameter to the discrimination of the individual laser surgeon.
EFFECTS ON BIOLOGICAL TISSUE
A laser beam’s effect on tissue will vary with the frequency of the laser light and the type of tissue with which the laser interacts. Two properties of the tissue are often used to describe this interaction: the coefficient of absorption and the selective absorption (American National Standards Institute [ANSI], 2005)
Coefficient of Absorption
The coefficient of absorption is a factor describing how rapidly the particular frequency of energy will be absorbed with depth. Low coefficients of absorption often correspond to very high percentages of scatter. If the energy is not well absorbed and is not highly reflected, it will attempt to transmit beyond the surface. As some laser energy penetrates, it allows for a large degree of scattering. Nd:YAG laser energy, in particular, scatters to a great degree, especially in tissue. In fact, scattering is the main factor that limits the penetration of the Nd:YAG laser in tissue. The absorption coefficient commonly given for 1064-nm wavelengths (Nd:YAG) in tissue are taking into account not only absorption but also scattering. Because “tissue” is not uniform in composition, these values will vary a great deal for different types of soft tissue and even for the same type of soft tissue from different locations (ANSI, 2005).
Selective Absorption
The selective absorption by pigmented tissues is the principal reason why the argon laser was initially so popular in ophthalmic surgery. The blue-green light energy from the argon laser transmits easily through the clear structures of the eye with minimal absorption by the lens and aqueous humor while being readily absorbed by the pigmented retina. Thus the laser energy is preferentially absorbed in the retinal area of the eye as is desired for performing many retinal procedures. The argon laser is now being replaced by the KTP 532-nm wavelength and diode laser technology. Similarly, both the KTP and Nd:YAG laser are extremely useful for the treatment of surface pigmented lesions, allowing laser energy to be absorbed primarily in the pigmented areas while sparing (relatively) the nonpigmented areas (Dennis, 2008).
Wavelength Versus Transmission
The far-infrared energy produced by the CO2 laser (wavelength = 10,600 nm) has extremely low transmission in soft tissue. Thus the CO2 laser energy is very well confined to the upper 0.1 mm of the tissue surface. The near-infrared energy produced by the Nd:YAG laser (wavelength = 1064 nm) penetrates deeper in nonpigmented soft tissue than the CO2 laser energy and scatters significantly. In fact, it is primarily the scattering by the tissue rather than absorption that limits the depth of penetration of Nd:YAG energy. The visible blue-green energy of the argon laser (wavelength = 488 nm) or the KTP (wavelength = 532 nm) would penetrate the deepest of the three lasers if the tissue were completely devoid of pigment and hemoglobin. However, the presence of pigment and blood, even in relatively avascular tissues, changes the depth of penetration drastically as a result of selective absorption. Therefore the depth of penetration by the argon or KTP laser energy is less than that of the Nd:YAG laser energy, and in extremely pigmented tissues the penetration depth can closely approximate that of the CO2 laser (ANSI, 2005).
Effects of Temperature on Tissue
Laser surgery is an accurate and efficient method of performing thermal surgery, in contrast to other modalities used in operating rooms. Of course, other considerations are the operator’s experience and understanding of the tool. The actual surgery results from the conversion of the laser energy into heat. In tissue, protein denaturation occurs at and above 60 ° C (140 ° F), which results in irreversible tissue injury. At 100 ° C (212 ° F), water converts into steam. At some point between 300 ° and 400 ° C (572 ° and 752 ° F), tissue will begin to carbonize, resulting in surgical smoke or plume. At approximately 530 ° C (986 ° F) and in the presence of oxygen, tissue will burn and evaporate. Although these temperature-related effects are similar for all medical lasers once the energy is converted to heat, the differences in absorption of the various wavelengths by the various components of tissue make a great difference in the actual heat conversion during laser surgery (ANSI, 2005).
Heat coagulation of tissue is generally a slow process until carbonization begins. When carbonization occurs, energy absorption increases as does the rise in surface temperature. This is true for all types of medical laser energy because carbon is such a good absorber in the infrared and visible portion of the electromagnetic spectrum. A laser beam should be activated continuously on carbonized tissue. This is particularly true for the CO2 laser, but also applies to the Nd:YAG, the diode 830 nm, the KTP, and the argon lasers. Carbon will absorb laser energy extremely well and will rapidly heat the surface of the tissue to 300 ° C to 500 ° C (572 ° to 932 ° F). Surface temperatures this high will significantly extend the zone of thermal necrosis underlying the tissue surface as thermal conductivity spreads to heat the adjacent structures (ANSI, 2005).
LASER SAFETY
Federal Regulatory Agencies and Nongovernmental Controls
The federal Performance Standards for Light-Emitting Products (21 CFR 1040.10) (U.S. Food and Drug Administration, U.S. Department of Health and Human Services, 2009) requires that the operating manuals from the manufacturers provide the following information: calibration, maintenance, warning signs, nominal hazard zone, specific laser eyewear, and safety instructions that the laser safety officer can incorporate into standard operating procedures.
FDA and the National Center for Devices and Radiological Health
All medical lasers are regulated by the U.S. Food and Drug Administration (FDA) under the Medical Device Amendments to the Food and Drug Act, which apply primarily to laser manufacturers. These regulations are enforced by the National Center for Devices and Radiological Health (NCDRH). The FDA regulates more than 250 types of lasers, including those intended for medical and surgical use. The federal guidelines require manufacturers to classify the medical laser systems based on the laser’s ability to cause damage to the eye and skin. They are categorized under FDA classification of medical devices as class III, subdivision class 4, because of the potential hazards. Manufacturers must conform to all safety requirements of the federal standard. They must also be approved by the NCDRH before any marketing or testing of a laser for a particular clinical application or use and must comply with the labeling requirements (ANSI, 2005).
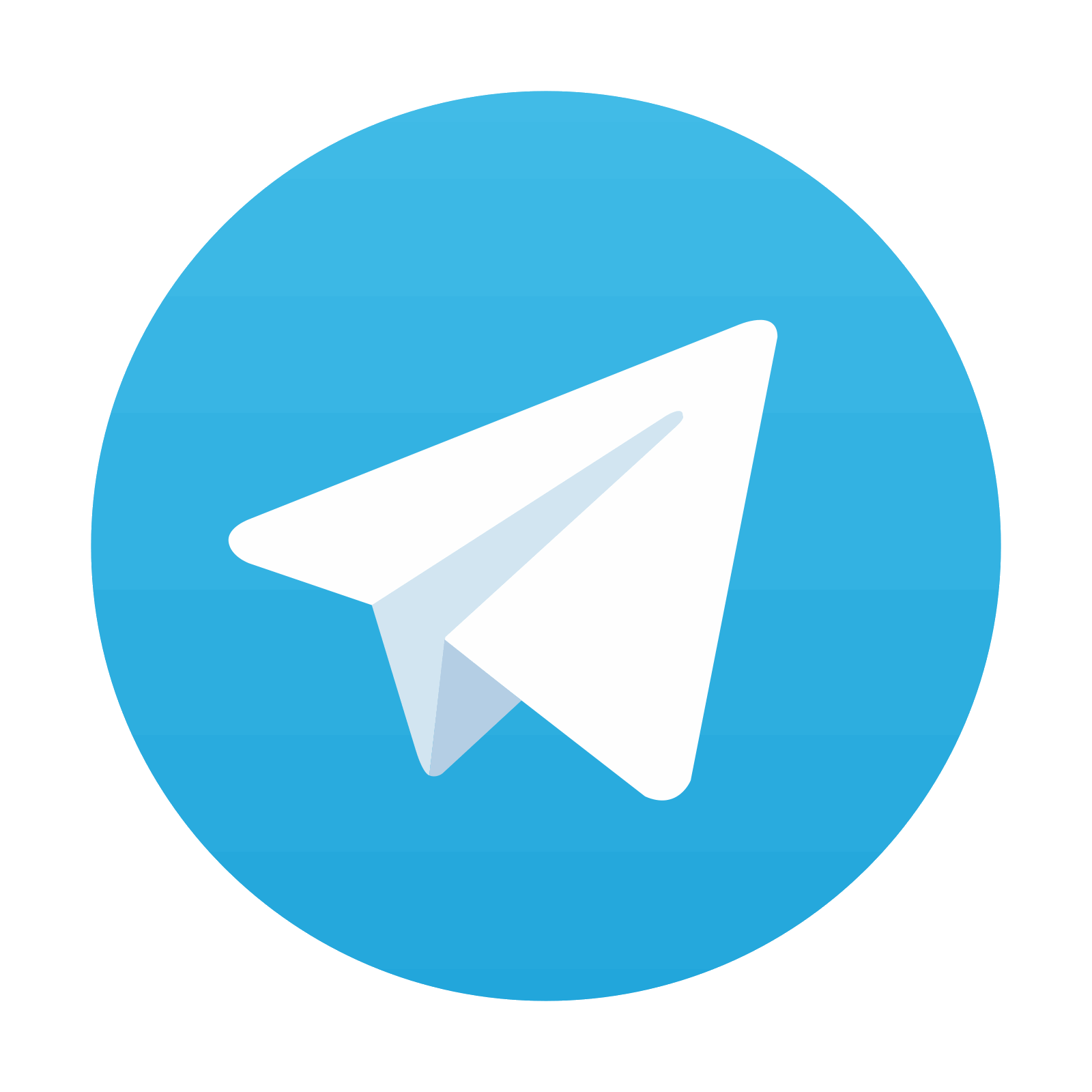
Stay updated, free articles. Join our Telegram channel
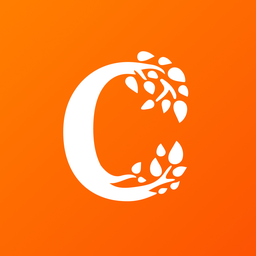
Full access? Get Clinical Tree
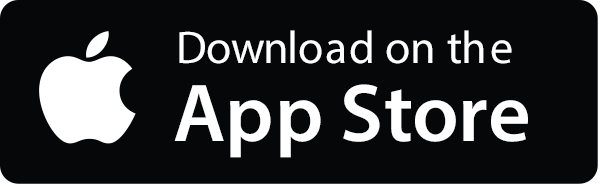
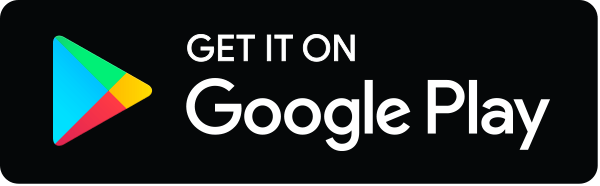