Abstract
Iron – like most micronutrients – is not synthesized by the human body and is acquired from dietary sources. Total body iron (approximately 3000–5000 mg) is chiefly regulated by varying the rate of absorption, balancing daily loss. Iron undergoes a continual cycle of conservation and re-utilization, with precise physiological controls to avoid systemic toxicity from free iron. Iron exerts pivotal roles in oxygen transport by haemoglobin, adenosine triphosphate generation, and enzymatic function. Iron deficiency anaemia is often identified in the preoperative period. Furthermore, syndromes of iron overload and subsequent toxicity may arise from aberrant pathways of iron metabolism.
After reading this article, you should be able to:
- •
understand the distribution of total body iron
- •
describe how iron is absorbed, cycled and stored
- •
understand the role of iron in haemoglobin
Iron is an essential micronutrient with several vital roles in oxygen transport, oxygen storage, enzymatic function, DNA synthesis and energy generation. The human body contains between 3000 mg and 5000 mg of iron. Sixty-five per cent of this total body iron (TBI) is found in haemoglobin, 10% in myoglobin (within muscle fibres) and other tissues, with the remainder stored within the liver, bone marrow and macrophages ( Figure 1 ). Iron homeostasis is a vital product of human evolution, with tight regulation of the uptake, transport, storage and export of iron an essential process to ensure the adequate balance of supply and demand, and avoiding situations of iron toxicity.

Sources of iron
Iron is not synthesized within the body and, therefore, must be absorbed from dietary sources. A typical adult diet contains 10–15 mg of elemental iron each day; of this only 7–20% is typically absorbed. The recommended daily allowance of iron is shown in Box 1 . Dietary sources of iron include haem iron from red meat, fish and poultry, and non-haem iron from vegetables and grains.
Iron handling
Iron absorption
There is no physiological mechanism by which an excess of total body iron may be excreted. Consequently, TBI is regulated through the precise control of dietary iron absorption, with the duodenum acting as the principle site of absorption. A loss of approximately 1–2 mg of iron occurs daily due to the sloughing of cells from the enteric mucosa, desquamation of skins cells, and from the formation of sweat and urine. This loss is typically matched by a daily absorption of 1–2 mg. As discussed, the quantity absorbed represents only a small proportion (7–20%) of the total daily dietary intake of iron. Physiological conditions that cause increased iron loss (e.g. menstruation, lactation) may be balanced with an absorption of up to 3 mg/day. Dietary iron is presented to the intestinal mucosa in the form of both haem iron and elemental (non-haem) iron. Haem iron is that which is contained within haemoglobin and myoglobin of animal tissue and is absorbed much more readily than elemental iron, although its exact mechanism of absorption is uncertain. , The haem carrier protein-1 (HCP-1) that had initially been thought to be the means of haem iron absorption into the duodenal enterocyte has subsequently been correctly identified as a folate transporter.
Elemental iron exists in two valency states – the reduced ferrous (Fe 2+ ) state, which is readily absorbable, and the oxidized ferric (Fe 3+ ) state, with limited solubility. Elemental iron can only be absorbed by the duodenal enterocytes in its ferrous state. Any elemental iron in the ferric state must be reduced by duodenal cytochrome b (also called cytochrome b reductase 1), which is present on the apical membrane of duodenal enterocytes. The resulting ferrous iron can then be taken into the cytoplasm of the duodenal cell by the divalent metal ion transporter-1 (DMT-1), located on the duodenal brush border. The uptake of elemental iron requires acidic conditions to aid the solubility of ferrous iron and to provide protons for co-transport via the DMT-1. This process is aided by the presence of dietary ascorbic acid, which is oxidized into dehydroascorbic acid and provides electrons to catalyse the reduction of ferric to ferrous iron. Once the ferrous iron has been absorbed into the enterocyte cytoplasm it may be utilized in one of three ways. Firstly, it may be transferred to local mitochondria to produce haem molecules. Secondly, it may be transferred into ferritin and then stored within the enterocyte, commonly when iron demand in the body is low. Thirdly, it may be removed from the cell to be transported to distant body sites. To be released as circulating iron, ferrous iron is exported from the enterocyte by the transmembrane receptor ferroportin (FPN), which is found on the basolateral membrane. This process involves the oxidization of Fe 2+ to Fe 3+ by haphaestin and caeruloplasmin, which can then be loaded onto the main plasma ion carrier transferrin. Ferroportin is also present on reticuloendothelial macrophages.
The rate of iron absorption in the duodenum and the release of iron to the circulation is regulated to precisely meet the systemic demands. The hormone hepcidin, a small peptide molecule released mainly from the liver, is chiefly responsible for this. Hepcidin is released when the body stores of iron are adequate or high, as detected by the liver iron stores and circulating iron levels. Its mechanism of action is to block iron release to the circulation by degrading the ferroportin transporter of both duodenal enterocytes and reticuloendothelial macrophages. Consequently, the iron these cells contain becomes sequestered within each cell. Enterocytes experience a rapid turnover; the iron sequestered within them will be lost as they are shed from the enteric mucosa, thereby reducing the duodenal absorption of iron. Hepcidin may further reduce duodenal iron absorption by impeding DMT-1 transcription at the enterocyte. Hepcidin is an acute phase protein that is also expressed under conditions of infection and systemic inflammation in response to cytokines, principally interleukin-6. , This host response to infection limits the amount of circulating iron available to any invading pathogen – to the potential benefit of the host. Through this inflammatory response, hepcidin is responsible for the iron sequestration seen in the anaemia of chronic disease. Hepcidin expression is down-regulated by increased red blood cell production (erythropoiesis), iron deficiency and tissue hypoxia.
The hepcidin–ferroportin (FPN) axis
Recent discoveries in hepcidin have provided insight into its role as the major regulatory hormone in iron homeostasis at the systemic level. Hepcidin concentration can vary in physiological states such as pregnancy, but its dysregulation can also contribute to pathological iron disorders of overload and deficiency. Hepcidin exerts its regulatory effects by binding to the transmembrane receptor ferroportin (FPN), which functions as an iron exporter and is found in duodenal enterocytes, macrophages and hepatocytes as described above. This binding leads to ubiquitination, internalization and degradation of FPN within lysosomes, inhibiting iron export and reducing circulating iron concentrations when hepcidin expression is stimulated.
Iron transfer mechanisms
Iron is a highly reactive element. Consequently, a system of organic molecules must bind free iron so that it can be transferred and stored without causing cellular damage from the formation of reactive oxygen species. Apotransferrin is a glycoprotein that binds free iron, forming the molecule transferrin, which is the means of transporting circulating iron in the plasma. The plasma iron concentration is minimal (3–4 mg) and must meet the demands of high turnover (20–25 mg) from erythropoiesis and other tissue demands. Transferrin plays a key role in this process and binds iron that is released to the systemic circulation from duodenal enterocytes and macrophages through binding to the TRF1 receptor. , Transferrin binds with less affinity to its TRF2 receptor but this process is now known to be key in iron homeostasis, causing upregulation of hepcidin and reduction of erythropoiesis responsiveness in iron overload, with the opposite in deficiency.
Transferrin supplies the erythroblasts in the bone marrow with circulating iron for erythropoiesis. It supplies the tissues that require iron for growth and reparative processes. It also supplies the iron that binds to the storage molecules ferritin and haemosiderin. Transferrin also plays an important role in the buffering of non-transferrin bound iron which can cause oxidative injury through the creation of reactive oxygen species (ROS) via the Fenton reaction.
The iron cycle
Total body iron undergoes a continual cycle of conservation and re-utilization. Although only 1–2 mg is absorbed each day, iron is continually released from the haem molecules of senescent reticulocytes that are broken down by phagocytosis in the spleen. The reticuloendothelial macrophages that perform this process turn over approximately 30 mg of iron daily. These iron-containing macrophages constitute a large, dynamic iron reservoir that is regulated by the hormone hepcidin.
Iron storage
Iron can be stored in its ferric (Fe 3+ ) state, bound to either ferritin or haemosiderin. Ferritin is a ubiquitous protein, common to all body cells. For this reason, every cell can store ferric iron intracellularly. The predominant storage sites are the liver (25%), spleen and bone marrow. Ferritin is a large protein molecule of 480 kDa with a maximum binding capacity of 4500 ferric iron atoms per ferritin molecule. However, each molecule of ferritin typically binds less than its maximal capacity (approximately 2000 ions). Ferritin is a soluble complex that is easily mobilized, and constitutes two-thirds of the stored iron within the liver. It mainly originates from macrophages and is a good correlation of body iron stores, however its value can be affected by many conditions including infection, inflammation and malignancy. One-third of iron stores within hepatocytes are bound to haemosiderin, which is a degraded form of ferritin and is relatively insoluble and slow to mobilize. Iron can also be incorporated into iron-sulphur cluster (Fe-s) proteins, and a small amount can be found in the circulation, bound to either transferrin or non-transferrin carriers such as citrate and albumin.
Iron and cytochromes
Iron plays a key role in oxidative phosphorylation and adenosine triphosphate (ATP) generation due to its presence in cytochromes. Cytochromes are haem-containing proteins that utilize electron transfer to alternately oxidize and reduce the iron component. In oxidative phosphorylation, the flow of electrons is coupled to ATP production.
Iron and haemoglobin
Iron is a key component of the haem moiety of the haemoglobin molecule. Haem consists of an organic portion (a protoporphyrin ring) surrounding a central iron ion (in its ferrous state, Fe 2+ ). The Fe 2+ ion forms six bonds in total within haem; four to nitrogen atoms with the protoprophyrin ring, one to a histidine residue on an α-globin chain and the final bond made to oxygen as needed. Haem is synthesized both in the cytosol and the mitochondria of erythrocytes. A haemoglobin molecule consists of four polypeptide globin chains (two α, two β in HbA) with each chain surrounding a haem moiety ( Figure 2 ); 95% adult haemoglobin is HbA. The main function of haemoglobin is the transport of oxygen from lungs to tissues. Its secondary functions include carbon dioxide carriage and buffering of hydrogen ions in the erythrocyte.
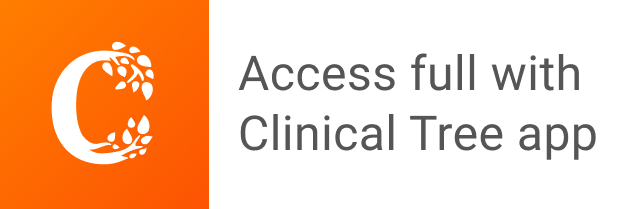