Abstract
This chapter reviews the basic and clinical pharmacology of intravenous opioids in the context of anesthesiology and perioperative medicine. Unique features of commonly used opioids are discussed and the principles underlying rational drug selection and administration are outlined. Emerging developments of contermporary interest are briefly examined.
Keywords
Opioids, morphine, fentanyl, remifentanil, codeine, naloxone, naltrexone, biased opioids, opioid epidemic
Historical Perspective
The white “latex” juice of the poppy plant is the source of more than 20 opiate alkaloids. Opium, a word derived from the Greek word for juice, is the brownish residue observed after the poppy’s juice is desiccated. Opiate is the older term classically used in pharmacology to mean a drug derived from opium. Opioid, a more modern term, is used to designate all substances, both natural and synthetic, that bind to opioid receptors (including antagonists).
Opium and its derivatives have been known for millennia to relieve pain. Laudanum, or tincture of opium (a mixture of opium and alcohol), was used as early as the 1600s as an analgesic. Sir Christopher Wren, the acclaimed Englishman of arts and letters, was the first to inject opium into a living organism using a hollow feather quill as the delivery system in 1659. Sertürner, a German pharmacist, isolated morphine from opium in 1805. Having initially called morphine “somniferum” (after the Latin botanical name Papaver somniferum — the poppy that brings sleep), the name was later changed to morphine, alluding to Morpheus, the Greek god of dreams.
Opium and its derivatives have sometimes been the basis of international conflict. The Opium Wars of the 1800s were fought between China and the Western powers in large part in response to Western importation of opium into China. The opium houses of 19th century China, where opium was freely available for sale and consumption, illustrated the frightening societal consequences of large-scale drug abuse. Recognition of these problems in the United States eventually culminated in the Harrison Narcotics Tax Act of 1914 that criminalized narcotic possession. The prevalence of addiction clinics and drug-related violence and crime in modern society emphasizes the chronicity of the societal difficulties that stem from this drug class and others. The ongoing opioid abuse epidemic in the United States is a recent reflection of the complexity and recalcitrance of the problems associated with opioid addiction despite nearly a century of research devoted to the conundrum.
Despite their abuse potential, opioids play an indispensable role in the practice of medicine, especially anesthesiology, critical care, and pain management. A sound understanding of opioid pharmacology, including both basic science and clinical aspects, is critical for the safe and effective use of these important drugs. This chapter focuses almost exclusively on intravenous opioids used perioperatively.
Basic Pharmacology
Structure-Activity
The opioids of clinical interest in anesthesiology share many structural features. Morphine, the principal active compound derived from opium, is a benzylisoquinoline alkaloid; the benzylisoquinoline structural backbone is present in many important naturally occurring drugs including papaverine, tubocurarine, and morphine. Morphine’s benzylisoquinoline based structure is shown in Fig. 17.1 . Many commonly used semisynthetic opioids are created by simple modification of the morphine molecule. Codeine, for example, is the 3-methyl derivative of morphine. Similarly, hydromorphone, hydrocodone, and oxycodone are also synthesized by relatively simple modifications of morphine. More complex alteration of the morphine molecular skeleton results in mixed agonist-antagonists such as nalbuphine and even pure competitive antagonists such as naloxone.

Some of the morphine derivatives have chiral centers and thus are typically synthesized as racemic mixtures of two enantiomers; only the levorotatory enantiomer is significantly active at the opioid receptor. The naturally occurring, stereospecific enzymatic machinery in the poppy plant produces morphine only in the levorotatory form.
The fentanyl series of opioids are chemically related to meperidine. Meperidine is the first completely synthetic opioid and can be regarded as the prototype clinical phenylpiperidine. As shown in Fig. 17.1 , fentanyl is a simple modification of the basic phenylpiperidine structure found within meperidine; the other commonly used fentanyl congeners such as alfentanil and sufentanil are somewhat more complex versions of the same phenylpiperidine skeleton. As these drugs have no chiral center and therefore exist in a single form, the pharmacologic complexities of stereochemistry do not apply.
As shown in Fig. 17.2 , evidence from x-ray crystallography investigations and other techniques suggests that distinct parts of the opioid agonist or antagonist molecule confer specificity (i.e., addressing) and efficacy (i.e., messaging). Agonists and antagonists have similar addressing segments in their structures; their action at the receptor is determined by the messaging portion of the molecule (see Chapter 1 ).

As outlined in Table 17.1 , opioids share many physicochemical features in common, although some individual drugs have unique features. In general, opioids are highly lipid soluble, weak bases that are highly protein bound and largely ionized (protonated) at physiologic pH. Opioid physicochemical properties are thought to have important implications on their clinical behavior. For example, highly lipid soluble, relatively unbound, un-ionized molecules such as alfentanil and remifentanil have a shorter latency to peak effect after bolus injection presumably because of their more rapid transfer across cellular membranes.
Parameter | Morphine | Fentanyl | Sufentanil | Alfentanil | Remifentanil |
---|---|---|---|---|---|
pKa | 8.0 | 8.4 | 8.0 | 6.5 | 7.1 |
% Un-ionized at pH 7.4 | 23 | <10 | 20 | 90 | 67? |
Octanol-H 2 O partition coefficient | 1.4 | 813 | 1778 | 145 | 17.9 |
% Bound to plasma protein | 20-40 | 84 | 93 | 92 | 80 |
Diffusible fraction (%) | 16.8 | 1.5 | 1.6 | 8.0 | 13.3 |
Vdc (L/kg) | 0.1-0.4 | 0.4-1.0 | 0.2 | 1.1-0.3 | 0.06-0.08 |
Vdss (L/kg) | 3-5 | 3-5 | 2.5-3.0 | 0.4-1.0 | 0.2-0.3 |
Clearance (mL/min per kilogram) | 15-30 | 10 | 10-15 | 4-9 | 30-40 |
Hepatic extraction ratio | 0.6-0.8 | 0.8-1.0 | 0.7-0.9 | 0.3-0.5 | NA |
Mechanism
Opioids produce their main pharmacologic effects by interacting with opioid receptors. Investigation of opioid receptor genetics, structure, and function over the past several decades has greatly enhanced understanding of opioid pharmacology.
Cloning of the opioid receptors, first accomplished in rodents and subsequently in humans, was an important first step in eventually elucidating opioid receptor structure and function. Using the rat µ-opioid receptor complementary DNA sequence as a guide, investigators were able to identify its human homolog, describing the amino acid sequence, strong binding affinity for an endogenous opioid ligand (i.e., enkephalin) and chromosomal assignment (i.e., chromosome 6).
Opioid receptors are typical of the G-protein–coupled family of receptors widely found in biology (e.g., β-adrenergic, dopaminergic, among others). Like other G-protein–coupled receptors, opioid receptors have seven transmembrane portions, intracellular and extracellular loops, an extracellular N-terminus, and an intracellular C-terminus. There is considerable amino acid sequence homology among the various opioid receptor subtypes; most of the nonhomologous sequences occur in the extracellular portions of the protein. The extracellular domains are thought to be important in discriminating between the various receptor ligands.
Expression of cloned opioid receptors in cultured cells has facilitated analysis of the intracellular signal transduction mechanisms activated by opioid receptors. As shown in Fig. 17.3 , binding of opioid agonists with the receptors leads to activation of the G-protein (actually three distinct G-protein subunits), producing effects that are primarily inhibitory (decreased cyclic adenosine monophosphate production, decreased calcium ion influx, increased potassium ion efflux); these effects ultimately culminate in membrane hyperpolarization of the cell and reduction of neuronal excitability.

Three classical opioid receptors have been identified using molecular biology techniques: µ, κ, and δ receptors. These receptors are also described by an international nomenclature scheme as MOP, KOP, and DOP (mu, kappa, or delta opioid peptide). The µ-opioid receptor is commonly abbreviated in the scientific literature as MOR. More recently, a fourth opioid receptor, ORL1 (also known as NOP), has also been identified, although its function appears to be quite different from the classical opioid receptors. As shown in Table 17.2 , each of these opioid receptors has a commonly used experimental bioassay, associated endogenous ligand(s), a set of agonists and antagonists, and a spectrum of physiologic effects when the receptor is activated. Although the existence of opioid receptor subtypes (e.g., µ-1, µ-2, and so on) has been proposed, it is not clear from molecular biology techniques that distinct genes code for them. Posttranscriptional and posttranslational modification of opioid receptors certainly occurs and could be responsible for conflicting data regarding opioid receptor subtypes. It is well known, for example, that the messenger RNA coding for the human µ-receptor undergoes alternative splicing, resulting in subtle differences in the final receptor.
TYPE OF RECEPTOR | |||
---|---|---|---|
Mu (µ) | Delta (δ) | Kappa (Κ) | |
Tissue bioassay a | Guinea pig ileum | Mouse vas deferens | Rabbit vas deferens |
Endogenous ligand | β-Endorphin | Leu-enkephalin | Dynorphin |
Endomorphin | Met-enkephalin | ||
Agonist prototype | Morphine | Deltorphin | Buprenorphine |
Fentanyl | Pentazocine | ||
Antagonist prototype | Naloxone | Naloxone | Naloxone |
Supraspinal analgesia | Yes | Yes | Yes |
Spinal anagesia | Yes | Yes | Yes |
Respiratory depression | Yes | No | No |
Gastrointestinal effects | Yes | No | Yes |
Sedation | Yes | No | Yes |
a Traditional experimental method to assess opioid receptor activity in vivo.
As confirmed by autoradiographic and binding techniques, opioid receptors are widely expressed in the central nervous system (CNS). In the spinal cord, they are mainly localized to the substantia gelatinosa of the dorsal horn. In the brain, they are especially concentrated in the periaqueductal gray region, the limbic system, area postrema, thalamus, and cerebral cortex. Opioid receptors are also found outside the CNS in gastrointestinal, biliary, and other tissues. Action by agonists at these peripheral receptors accounts for some of the adverse effects of opioids such as constipation.
In accordance with the wide distribution of opioid receptors in the CNS, opioids appear to exert their therapeutic effects at multiple sites. Opioid agonism at the level of the spinal cord inhibits the release of substance P from primary sensory neurons in the dorsal horn, mitigating the transfer of painful sensations to the brain. Opioid actions in the brainstem modulate nociceptive transmission in the dorsal horn of the spinal cord through descending inhibitory pathways. Opioids are also thought to change the affective response to pain through actions in the forebrain; decerebration prevents opioid analgesic efficacy in rats. Furthermore, functional magnetic resonance imaging studies in humans have demonstrated that morphine induces signal activity in reward structures in the brain.
Studies in genetically altered mice have yielded important information about opioid receptor function. In µ-opioid receptor knockout mice, morphine induced analgesia, reward effect, and withdrawal effect are absent. Fig. 17.4 demonstrates the simple power of these site-directed mutagenesis techniques, confirming how MOR knockout mice exhibit no analgesic effect to morphine as assessed by measuring the time to jumping off a hot plate. Importantly, MOR knockout mice also fail to exhibit respiratory depression in response to morphine. Confirming the importance of opioid receptor ligands in the biology of “endogenous” analgesia systems, β-endorphin knockout mice fail to show naloxone-reversible, stress-induced analgesia but maintain a normal analgesic response to morphine.

Opioid addiction, as with many addictive drugs and practices, involves dopamine-related reward mechanisms. As shown in Fig. 17.5 , microdialysis techniques in a rodent model demonstrate an opioid-induced, naloxone-reversible increase in dopamine levels in the nucleus accumbens region, a brain area known to be important in addiction (see Chapter 12 ). Toll-like receptors (i.e., TLR4), a receptor system involved in the identification of foreign molecules and the inflammatory response in the CNS, may play a role in the biology of opioid addiction; in silico evidence confirms that opioids such as remifentanil can interact with this receptor complex.

Metabolism
The intravenous opioids in common perioperative clinical use are transformed and excreted by a wide variety of metabolic pathways. In general, opioids are metabolized by the hepatic microsomal cytochrome P450 (CYP) system, although hepatic conjugation and subsequent excretion by the kidney are important for some drugs. The major and minor metabolic enzymes involved in the metabolism of a number of commonly used opioids are shown in Fig. 17.6 . For certain opioids, the specific metabolic pathway involved has important clinical implications in terms of active or toxic metabolites (e.g., morphine, meperidine) or an ultrashort duration of action (e.g., remifentanil). For others opioids, genetic variation in the metabolic pathway can drastically alter the clinical effects (e.g., codeine). These nuances are addressed in a subsequent section focused on individual drugs.

Clinical Pharmacology
Pharmacokinetics
Because in most respects opioids are pharmacodynamically similar, pharmacokinetic differences form the scientific foundation for the rational selection and administration of opioids in anesthesia practice. Key pharmacokinetic behaviors to consider are the latency-to-peak effect after bolus injection (i.e., bolus front-end kinetics), the time to offset of effect after bolus injection (i.e., bolus back-end kinetics), the time to steady-state after starting a continuous infusion (i.e., infusion front-end kinetics), and the time to offset of effect after stopping a continuous infusion (i.e., infusion back-end kinetics).
Applying opioid pharmacokinetic concepts to clinical anesthesiology requires recognition of several fundamental principles. First, a table of opioid pharmacokinetic parameters can rarely be instructive in terms of understanding how the pharmacokinetic behavior of the individual drugs compares (see Chapter 2 ). Understanding pharmacokinetic behavior is best achieved through computer simulation. Second, because opioid clinical behavior differs depending on whether the drugs are administered by bolus injection or continuous infusion, these two clinical conditions must be considered separately. Third, the time course of drug levels predicted through pharmacokinetic simulation cannot be considered in a vacuum; pharmacokinetic information must be integrated with knowledge about the concentration-effect relationship and drug interactions (pharmacodynamics) to be clinically useful.
The latency-to-peak effect and the offset of effect after bolus injection of various opioids can be explored by predicting the time course of effect site concentrations after a bolus is administered. Because the opioids differ in terms of potency (and thus the required dosages), for comparison purposes the effect site concentrations must be normalized to the percent of peak concentration for each drug. Considering morphine, hydromorphone, fentanyl, sufentanil, alfentanil, and remifentanil as among the most commonly used intravenous opioids intraoperatively, the top panel of Fig. 17.7 illustrates how these opioids differ in terms of latency-to-peak effect (i.e., bolus front-end kinetics); the simulations are based on pharmacokinetic-dynamic parameters from the literature.

The simulation of a bolus injection ( Fig. 17.7A ) has obvious clinical implications. For example, when a rapid onset of opioid effect is desirable, morphine may not be a good choice. Similarly, when the clinical goal is a brief pulse of opioid effect followed by a rapid dissipation of effect, remifentanil or alfentanil is preferred. Note how remifentanil’s concentration has declined substantially before fentanyl’s peak concentration has been achieved (bolus back-end kinetics). The simulation illustrates why the front-end kinetics of fentanyl make it a drug well suited for patient-controlled analgesia (PCA). In contrast to morphine, the peak effect of a fentanyl bolus is manifest before a typical PCA lockout period has elapsed, thus mitigating a “dose stacking” problem.
The latency-to-peak effect is governed by the speed with which the plasma and effect site come to equilibrium (the k eo parameter). Drugs with a more rapid equilibration are thought to have a higher “diffusible” fraction (the proportion of drug that is un-ionized and unbound). It is important to emphasize that a substantial limitation of these latency-to-peak effect simulations is that a very large dose of any opioid can produce a rapid onset (because a supratherapeutic drug level in the effect site is reached even though the peak concentration comes later).
The time to steady-state after beginning a continuous infusion is also best explored through pharmacokinetic simulation. Again, because the potency of the various opioids differs, the predicted effect site concentrations must be normalized to the final steady-state concentrations to contrast the behavior of the drugs. Using the same prototypes, Fig. 17.7B shows the time required to achieve steady-state effect site concentration (infusion front-end kinetics).
The simulation of simple, constant rate infusions has obvious clinical implications. First, it is clear that for most commonly used intravenous opioids, the time required to reach a substantial fraction of the ultimate steady-state concentration is very long in the context of intraoperative use. To reach a near steady-state more quickly requires a bolus be administered before the infusion is commenced (or increased). Remifentanil perhaps represents a partial exception to this general rule. Another extremely important clinical application of this simulation is that concentrations will rise for many hours after an infusion is commenced (without a bolus); thus practitioners must be aware that concentrations are typically rising even though the infusion rate may have been the same for hours. That remifentanil achieves a near steady-state relatively quickly makes it an especially useful drug for total intravenous anesthesia because it is thus more “titratable.”
The time to offset of effect after stopping a steady-state infusion is often explored through context-sensitive half-time simulation (CSHT). Defined as the time required to achieve a 50% decrease in plasma concentration after stopping a continuous, steady-state infusion, the CSHT is a means of normalizing the pharmacokinetic behavior of drugs so that rational comparisons can be made regarding the predicted offset of drug effect. The CSHT is thus focused on infusion back-end kinetics.
Fig. 17.7C shows a CSHT simulation for commonly used opioids (strictly speaking, it is the 50% decrement time because the simulation is for effect site concentrations). Several clinically important points are obvious. First, for most drugs, the time to a 50% decrease in concentration changes with the length of the infusion. Thus for very brief infusions, the predicted back-end kinetics for the various drugs do not differ much (remifentanil is a notable exception). As the infusion time lengthens, the CSHTs begin to differentiate, providing a rational basis for drug selection. Second, depending on the desired duration of opioid effect, the clinician can choose the shorter-acting drugs or the longer-acting ones. Finally, the shapes of these curves differ depending on the degree of concentration decline required; in other words, the curves representing the time required to achieve a 20% or an 80% decrease in concentration (e.g., the 20% or 80% decrement time simulations) are quite different. Thus, depending on the anesthesia technique applied, the CSHT simulations (or 50% decrement time) are not necessarily the clinically relevant simulations. It is also important to recognize that the CSHT simulation for morphine does not account for its active metabolite (see the section on individual drugs in later text).
Pharmacodynamics
Given that µ-receptor agonists share a common mechanism of action, in most respects the commonly used MOR agonists can be considered pharmacodynamic equals with important pharmacokinetic differences. In other words, their effect profiles, both therapeutic and adverse, are very similar. Their efficacy as analgesics and their propensity to produce ventilatory depression are indistinguishable when one µ-agonists is compared with another at equipotent concentrations. Where pharmacodynamic differences exist, they are typically a function of non-opioid receptor mechanisms, such as histamine release, among others.
Clinically important µ-agonist pharmacodynamic effects are observed in many organ systems. Fig. 17.8 summarizes the major pharmacodynamic effects of the fentanyl congeners. Depending on the clinical circumstances and clinical goals of treatment, some of these widespread effects can be viewed as therapeutic or adverse. For example, in some clinical settings the sedation produced by µ-agonists might be viewed as a goal of therapy; in others, this would clearly be thought of as an adverse effect.

Therapeutic Effects
The relief of pain is the primary therapeutic effect of opioid analgesics. Acting at spinal and brain µ-receptors, opioids are thought to provide analgesia both by attenuating the nociceptive traffic from the periphery and also by altering the affective response to painful stimulation centrally. µ-agonists are most effective in treating “second pain” sensations carried by slowly conducting, unmyelinated C fibers; they are less effective in treating “first pain” sensations (carried by small, myelinated A-δ fibers) and neuropathic pain. A unique aspect of opioid-induced analgesia (in contrast to drugs like local anesthetics) is that other sensory modalities are not affected (e.g., touch, temperature, among others).
Perioperatively (certainly intraoperatively), the sedation produced by µ-agonists is also one of the targeted effects. The brain is the anatomic substrate for the sedative action of µ-agonists. With increasing doses, µ-agonists eventually produce drowsiness and sleep (the relief of pain no doubt contributes to the promotion of sleep in uncomfortable patients both preoperatively and postoperatively, independent of the intrinsic sedative effect). With sufficient doses (higher than typically used clinically), the µ-agonists produce pronounced δ wave activity on the electroencephalogram that resembles the pattern observed during natural sleep.
µ-agonists produce significant pain relief at doses that do not produce sleep; this is the basis of their clinical utility in the treatment of pain in ambulatory patients. On the other hand, the fact that increasing doses eventually produce drowsiness (and therefore the inability to request additional doses) is the essential scientific foundation for the safety of patient controlled analgesia devices. It is important to emphasize that µ-agonists, even when given in very high doses, do not reliably produce unresponsiveness and amnesia; thus they cannot be viewed as complete anesthetics when used alone.
Suppression of the cough reflex is another important therapeutic effect of the µ-agonists in the practice of anesthesia. This antitussive effect is mediated through the cough centers in the medulla. Attenuation of the cough reflex presumably makes coughing and struggling against an indwelling endotracheal tube less likely. This effect is particularly important during fiberoptic intubation of the trachea in an awake patient as an approach to the difficult airway. Curiously, dextrorotatory isomers of opioids (e.g., dextromethorphan) produce this effect even though they do not have analgesic activity. Opioids can sometimes be associated with a paradoxical increase in coughing, typically after a bolus dose is administered. This poorly understood phenomenon is often briefly observed during induction of anesthesia when opioids are used as part of the induction regimen.
Adverse Effects
Depression of ventilation is the most significant adverse effect associated with µ-agonist drugs. Of course, when the airway is secured and ventilation is controlled intraoperatively, opioid-induced depression of ventilation is of little consequence. However, in the postoperative period, significant depression of ventilation associated with opioid analgesia can be a life-threatening complication and is a major source of morbidity and mortality, especially in high-risk populations such as patients with obstructive sleep apnea.
µ-agonists alter the ventilatory response to arterial carbon dioxide. That depression of ventilation is mediated by the MOR is supported by the observation that µ-receptor knockout mice do not exhibit the effect when they receive morphine. Central pattern generators in the brainstem are the main anatomic substrate for depression of ventilation by the µ-agonists (see Chapter 29 ). Detailed studies of the brainstem anatomy involved in the control of ventilation reveal multiple, anatomically proximate collections of cells that are responsive to opioid agonists and antagonists, including the pre-Bötzinger complex in the rostral medulla, and the parabrachial nucleus and Kölliker-Fuse nucleus in the rostral pons. An important nuance of these investigations is that these brainstem nuclei control not only the ventilatory pattern, but also influence the muscles that maintain airway patency.
The depression of ventilation is studied by artificially increasing inspired carbon dioxide while maintaining normal oxygen tension. As shown in Fig. 17.9 , an increase in arterial carbon dioxide partial pressure dramatically increases the minute ventilation. Under the influence of opioid analgesics, the curve is flattened and shifted to the right such that at a given carbon dioxide partial pressure the minute ventilation is lower. More importantly, the “hockey-stick” shape of the normal curve is lost such that under the influence of µ-agonists there may be a partial pressure of carbon dioxide below which the patient does not breathe (this point can be thought of as the “apneic threshold”). µ-agonists also depress the hypoxic drive to breathe, although this effect is less important clinically than the effect on carbon dioxide–controlled ventilatory drive.

The clinical signs of depressed ventilation are quite subtle with moderate opioid doses. Postoperative patients receiving opioid analgesic therapy can be awake and alert and yet sensitive testing will reveal significantly decreased minute ventilation. Moderate doses of µ-agonists produce a decrease in the ventilatory rate (often associated with a slightly increased tidal volume). As the opioid concentration is increased, both ventilatory rate and tidal volume progressively decrease, eventually culminating in an irregular ventilatory rhythm and then complete apnea.
A variety of factors can increase the risk of opioid-induced ventilatory depression ( Table 17.3 ). Clear risk factors include high opioid dose, advanced age, concomitant use of other CNS depressants, and renal insufficiency (for morphine). Natural sleep also appears to increase the ventilatory depressant effect of µ-agonists.
|
Opioids can alter cardiovascular physiology by a variety of mechanisms. Compared with many anesthetic drugs (e.g., propofol, volatile anesthetics), however, the cardiovascular effects of opioids, particularly the fentanyl congeners, are relatively mild (the unique cardiovascular effects of morphine and meperidine are discussed in the section on individual drugs). In fact, in some clinical circumstances, such as when anesthetizing patients with ischemic heart disease, the cardiovascular effects of the fentanyl congeners can be viewed as therapeutic.
The fentanyl congeners produce a slowing of heart rate by directly increasing vagal nerve tone in the brainstem. In experimental animals this effect can be blocked by microinjection of naloxone into the vagal nerve nucleus or by peripheral vagotomy. If the bradycardia is considered undesirable in patients, it can be readily treated with antimuscarinic drugs.
Opioids also produce vasodilation by depressing vasomotor centers in the brainstem (i.e., by decreasing central vasomotor tone) and, to a lesser extent, by a direct effect on vessels. This action affects both the venous and arterial vasculature, thereby reducing both preload and afterload. The resulting decrease in blood pressure, while typically mild in healthy patients, is much more pronounced in patients with elevated sympathetic tone such as patients with congestive heart failure or hypertension. At typical clinical doses, opioids do not appreciably alter myocardial contractility.
Opioid-induced muscle rigidity is another potentially serious adverse effect of this drug class. Typically observed during the rapid administration of high bolus doses of the fentanyl congeners, opioid-induced muscle rigidity can make bag-mask ventilation during anesthesia induction impossible; the difficulty with bag-mask ventilation appears to be in large part a function of vocal cord rigidity and closure. The rigidity tends to coincide with the onset of unresponsiveness. Although the mechanism of opioid-induced muscle rigidity is unknown, it does not appear to be a direct action on muscle fibers because it can be prevented and treated with neuromuscular blockers.
In the perioperative setting, perhaps one of the most vexing (although not life-threatening) adverse effects associated with the µ-agonists is nausea and vomiting. Opioids stimulate the chemoreceptor trigger zone in the area postrema on the floor of the fourth ventricle (opioid receptors are expressed here) in the brain. This can lead to nausea and vomiting, an effect that is exacerbated by movement (this is perhaps why ambulatory surgery patients are more likely to be troubled by postoperative nausea and vomiting [PONV]). The use of postoperative opioids is a clear risk factor for the occurrence of PONV. Numerous effective therapies exist for both the prophylaxis and treatment of PONV associated with opioids and other risk factors (see Chapter 28 ).
Although it is of no clinical consequence in terms of patient safety or suffering, the pupillary constriction induced by µ-agonists can be a useful diagnostic sign indicating some ongoing opioid effect. Opioids stimulate the Edinger-Westphal nucleus of the oculomotor nerve to produce miosis. Even small doses of opioid elicit the response and very little tolerance to the effect develops. Thus miosis is a useful albeit nonspecific indicator of opioid exposure even in opioid-tolerant patients. Opioid-induced pupillary constriction is naloxone reversible.
The µ-agonists have important effects on gastrointestinal physiology. Opioid receptors are found throughout the enteric plexus of the bowel; their activation causes tonic contraction of gastrointestinal smooth muscle, thereby decreasing coordinated, peristaltic contractions. Clinically, this results in delayed gastric emptying and presumably higher gastric volumes in patients receiving opioid therapy preoperatively. Postoperatively, patients can develop opioid-induced ileus that can potentially delay the resumption of proper nutrition and discharge from the hospital. An extension of this acute problem is the chronic constipation associated with long-term opioid therapy.
Similar effects are observed in the biliary system, which also has an abundance of µ-receptors. The µ-agonists can produce contraction of the gallbladder smooth muscle and spasm of the sphincter of Oddi, potentially causing a falsely positive cholangiogram during gallbladder and bile duct surgery. These untoward effects are completely reversible by naloxone and can be partially reversed by glucagon treatment. The mixed agonist-antagonists (e.g., butorphanol) appear to produce less pronounced biliary effects.
In contrast to the gastrointestinal system, the urologic effects of opioids are regarded as minimal. Opioids can cause urinary retention by decreasing bladder detrusor tone and by increasing the tone of the urinary sphincter. These effects are at least in part centrally mediated, although peripheral effects are also likely given the widespread presence of opioid receptors in the genitourinary tract. Although the urinary retention associated with opioid therapy is not typically pronounced, it can be troublesome in males, particularly when the opioid is administered intrathecally or epidurally.
The euphoria produced by µ-agonists must be viewed as an adverse effect because it is the primary reason this drug class is so widely abused. Opioid addicts experience a euphoria that drives a drug-seeking lifestyle that often ends tragically in death or incarceration. The physical tolerance and dependence that inevitably develop in chronic users result in an increased dose requirement and a withdrawal syndrome upon cessation of drug intake. The withdrawal syndrome is characterized by intense drug craving, restlessness, body aches and pains, runny nose, lacrimation, and perspiration. An acute withdrawal syndrome can be precipitated by the administration of opioid antagonists (e.g., naloxone) during routine clinical care to patients who are not openly known to be opioid addicts.
Although perhaps not typically thought of as an acute effect that would be relevant to perioperative practice, opioids are known to depress cellular immunity. This effect is particularly well documented for morphine, although data exist for other µ-agonists as well. Morphine and the endogenous opioid β-endorphin, for example, inhibit the transcription of interleukin 2 in activated T cells, among other immunologic effects. It appears that individual opioids (and perhaps classes of opioids) differ in terms of the exact nature and extent of their immunomodulatory effects. The clinical implications of opioid-induced impairment of cellular immunity are not well understood, but these effects might influence wound healing, perioperative infection risk, and cancer recurrence, among other outcomes. How this rapidly expanding knowledge will eventually impact clinical practice is evolving.
Drug Interactions
Drug interactions can be based on two mechanisms: pharmacokinetic (i.e., where one drug influences the concentration of the other) or pharmacodynamic (i.e., where one drug influences the effect of the other). In anesthesia practice, although unintended pharmacokinetic interactions sometimes occur, pharmacodynamic interactions occur with virtually every anesthetic and are produced by design.
The most common pharmacokinetic interaction in opioid clinical pharmacology is observed when intravenous opioids are combined with propofol. Perhaps because of the hemodynamic changes induced by propofol and their impact on pharmacokinetic processes, opioid concentrations are expected to be modestly higher when given in combination with a continuous propofol infusion.
The most important pharmacodynamic drug interaction involving opioids is the synergistic interaction that occurs when opioids are combined with CNS depressants (see Chapter 5 ). As illustrated in Fig. 17.10 for fentanyl and isoflurane, opioids dramatically reduce the minimum alveolar concentration (MAC) of a volatile anesthetic. Careful examination of “opioid-MAC reduction” data such as in Fig. 17.10 reveals several clinically critical concepts. First, opioids synergistically reduce MAC. Second, the MAC reduction is substantial (depending on the dose, as much as 75% or more). Third, most of the MAC reduction occurs at moderate opioid levels (i.e., even modest opioid doses substantially reduce MAC). Fourth, the MAC reduction is not complete; that is, opioids are not complete anesthetics. The addition of the opioid cannot completely eliminate the need for the other anesthetic. And fifth, there are an infinite number of hypnotic-opioid combinations that will achieve MAC (this implies that clinicians must choose the optimal combination based on the goals of the anesthetic and operation). As one might expect based on first principles, the synergistic interaction applies to both therapeutic and adverse effects such as depression of ventilation and hemodynamic variables.
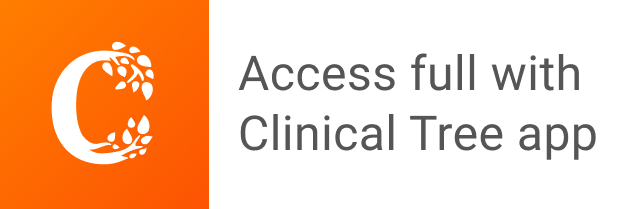