Key Points
- ▪
The introduction of thiopental into clinical practice in 1934 marked the beginning of modern intravenous (IV) anesthesia. Today, IV anesthetics are used for induction and maintenance of anesthesia and sedation in a wide variety of circumstances.
- ▪
The most commonly used IV anesthetic is propofol, an alkylphenol presently formulated in a lipid emulsion. Propofol provides rapid onset and offset with context-sensitive decrement times of approximately 10 minutes when infused for less than 3 hours and less than 40 minutes when infused for up to 8 hours. Its mechanism of action is likely the enhancement of gamma-aminobutyric acid (GABA)-induced chloride currents. Propofol causes a dose-dependent decrease in arterial blood pressure predominantly through a decrease in systemic vascular resistance and causes moderate respiratory depression. A unique action of propofol is its antiemetic effect, even at concentrations less than those producing sedation. A growing body of evidence suggests propofol may have antitumor potential.
- ▪
Barbiturates were the most commonly used IV drugs administered to induce anesthesia prior to the introduction of propofol. Thiopental provides rapid onset and offset when used as a single dose, but it accumulates rapidly with repeated or prolonged administration thus postponing recovery from anesthesia. Methohexital has a rapid onset and offset similar to propofol for procedures lasting less than 2 hours. The barbiturates are administered as sodium salts diluted in a water base at an alkaline pH. Similar to propofol, the barbiturates provide their hypnotic effects largely through action on the GABAA receptor. Barbiturates provide cerebral protection and are, apart from induction of anesthesia, used primarily for this purpose. They cause a moderate dose-dependent decrease in arterial blood pressure (primarily as a result of peripheral vasodilation) and respiratory drive. The barbiturates are contraindicated in patients with porphyria.
- ▪
The benzodiazepines are used primarily for anxiolysis and amnesia or for conscious sedation. The water-soluble benzodiazepine midazolam is most frequently used intravenously because of its rapid onset and offset compared with other benzodiazepines (e.g., diazepam). The onset time of midazolam is slower than that of propofol and barbiturates, and its offset, especially with larger doses or a prolonged infusion, is considerably longer than that of propofol or methohexital and may be prolonged in hepatic and renal failure. The benzodiazepines act through the GABA receptor. Flumazenil is a specific benzodiazepine antagonist. It can be used to reverse the effects of benzodiazepines but should be used with caution because its antagonizing effect often lasts shorter than the benzodiazepine effect that it is supposed to antagonize. The benzodiazepines generally produce only a mild decrease in arterial blood pressure and mild-to-moderate respiratory depression. Remimazolam is the most recent benzodiazepine with an ultrashort duration of action due to its rapid clearance through plasma esterases.
- ▪
Ketamine is a phencyclidine derivative that acts primarily, but not entirely, as antagonist of the N-methyl- d -aspartate (NMDA) receptor. It produces a dissociative state of hypnosis and analgesia. It has been used for induction and maintenance of anesthesia. Ketamine is associated with significant adverse psychological effects from larger doses and has several other side effects. It is used now primarily for its analgesic properties. It has rapid onset and relatively rapid offset, even after an infusion of several hours. It has sympathomimetic effects that preserve cardiac function. Ketamine has minimal effect on respiration and tends to preserve autonomic reflexes. In addition, ongoing research suggests ketamine may play a role as an antidepressant.
- ▪
Etomidate is an imidazole derivative used primarily for induction of anesthesia, especially in elderly patients and patients who are cardiovascularly compromised. It has a rapid onset of effect and a rapid offset even after a continuous infusion. A dose used to induce anesthesia results in inhibition of adrenocortical synthesis and possible mortality in intensive care unit (ICU) patients. The major advantage of etomidate is its minimal effect on the cardiovascular and respiratory systems.
- ▪
Dexmedetomidine is the most recently released IV anesthetic. It is a highly selective α 2 -adrenergic agonist that produces sedation, sympatholysis, hypnosis, and analgesia. Dexmedetomidine is approved for ICU sedation of initially intubated and mechanically ventilated patients, up to 24 hours. It may be advantageous for its ability to prevent delirium. Its second indication is procedural sedation of nonintubated patients. With increasing frequency dexmedetomine finds its use as a sedative during invasive or radiological procedures and as an adjunct in central or peripheral neural blockade. Its primary action is as an agonist on α 2 -receptors in the locus caeruleus. It has minimal effect on respiration. Heart rate and cardiac output show a concentration-dependent decrease.
- ▪
Droperidol, a butyrophenone and major tranquilizer, was initially used to produce a state of neuroleptanesthesia. Its prolongation of the QT interval has resulted in its withdrawal in several countries and its limitation to the treatment of postoperative nausea and vomiting (PONV) with a black box warning in the United States. Because the use of low-dose droperidol (<1.25 mg) for PONV has not been approved by the US Food and Drug Administration (FDA), the black box warning does not relate to this use. Clinically significant prolongation of the QT interval by doses used for PONV (0.625 to 1.25 mg) has been challenged by several editorials, and this effect has not been substantiated by review of the reported cases or other literature. Low-dose droperidol remains an effective antiemetic therapy and is used as such in many European countries (also see Chapter 80 ).
Acknowledgment
The editors and publisher would like to thank Drs. J.G. Reeves, Peter S.A. Glass, David A. Lubarsky, Matthew D. McEvoy, and Richardo Martinez-Ruiz for contributing a chapter on this topic in a previous edition of this work. It has served as the foundation for the current chapter.
Intravenous (IV) anesthesia can be traced back to 1656 with Percival Christopher Wren’s and Daniel Johann Major’s first experiments with the IV using a goose quill and bladder to inject wine and ale into a dog’s vein. In 1665 German naturalist and physician Sigismund Elsholz made the first attempt at IV anesthesia in humans and investigated the possibilities of IV injection with opiates. IV anesthesia further evolved when Fedoroff started using hedonal in St. Petersburg in 1905 and entered the era of modern anesthesia with the release of thiopental in 1936. Since these beginnings, and in particular during the past three decades, the pharmacokinetics and pharmacodynamics of IV anesthetics and their interactions have been described in increasingly greater detail. This body of knowledge and the availability of increasingly shorter-acting drugs now allow the anesthesia provider to administer anesthesia not on the basis of the needs of the population but to focus anesthesia on the individual needs of the patient. Today’s anesthesia provider is supported by modern IV drug administration techniques like target-controlled infusion and central nervous system (CNS) monitoring devices to further optimize and individualize the application of IV anesthesia. This chapter describes the current status of the pharmacology of IV anesthetics and their place in modern anesthesia.
Propofol
History
Since its introduction in the 1970s, propofol has become the most used IV hypnotic today. Building on work on the sedative properties of phenol derivates in mice, propofol was developed in the United Kingdom by Imperial Chemical Industries as ICI 35868. The initial solution of propofol, as released in 1977 in Cremophor EL, was withdrawn because of anaphylactic reactions, and replaced and reformulated as an emulsion of a soya oil/propofol mixture in water and relaunched in 1986. Propofol is used for induction and maintenance of anesthesia and for sedation in and outside the operating room.
Physicochemical Characteristics
Propofol ( Fig. 23.1 ) is one of a group of alkylphenols that were explored for their hypnotic properties in animals. The alkylphenols are highly lipid soluble and are insoluble in an aqueous solution. Numerous formulations of propofol are marketed today. The formulation most used is that of 1% propofol, 10% soybean oil, and 1.2% purified egg phospholipid added as emulsifier, with 2.25% of glycerol as a tonicity-adjusting agent, and sodium hydroxide to change the pH. Following concerns regarding microbial growth in the emulsion, EDTA was added for its bacteriostatic activities. Propofol has a pH of 7 and appears as a slightly viscous, milky white substance, a result of small lipid droplets in solution. In Europe, a 2% formulation and a formulation in which the emulsion contains a mixture of medium-chain and long-chain triglycerides also are available. All formulations commercially available are stable at room temperature, are not light sensitive, and may be diluted with 5% dextrose in water. Propofol concentrations may be measured both in whole blood and in the exhaled air.

In December 2008 the US Food and Drug Administration (FDA) approved fospropofol disodium (Lusedra) for monitored anesthesia care in adult patients undergoing diagnostic and therapeutic procedures. Fospropofol is a water-soluble prodrug of propofol that is metabolized by alkaline phosphatases in the liver to the active metabolite propofol. One millimole (mmol) of propofol is generated for each mmol of fospropofol sodium administered. About 1.86 mg of fospropofol sodium is the molar equivalent of 1 mg propofol. In April 2010, six studies on the pharmacokinetics and pharmacodynamics of fospropofol were retracted as a result of an analytical assay inaccuracy that was discovered after publication of these studies. Since then, few data on the pharmacokinetics and pharmacodynamics of fospropofol have been published. Although fospropofol remains available for monitored anesthesia care, data now available are scarce and most pharmacokinetic-pharmacodynamic data that are available come from the United States as described in a recent review. In contrast to propofol, fospropofol is not associated with pain on injection, although mild to moderate perineal paresthesias and pruritis minutes after a bolus injection of fospropofol have been reported and may be due to a phosphate metabolite.
Pharmacokinetics
Propofol is oxidized to 1,4-diisopropyl quinol in the liver. Propofol and 1,4-diisopropyl quinol are conjugated with glucuronic acid to propofol-1-glucuronide, quinol-1-glucuronide, and quinol-4-glucuronide, which then may be excreted by the kidneys. After a 2.5-hour anesthetic with propofol, patients excrete propofol and propofol metabolites for over 60 hours. Less than 1% propofol is excreted unchanged in urine, and only 2% is excreted in feces. The metabolites of propofol are thought to be inactive. Because clearance of propofol (>1.5 L/min) exceeds hepatic blood flow, extrahepatic metabolism or extrarenal elimination may occur. Extrahepatic metabolism has been confirmed during the anhepatic phase of patients receiving a transplanted liver with the determination of propofol metabolites after propofol administration in the absence of liver tissue. The most important extrahepatic site for propofol metabolism is the kidney. Renal metabolism of propofol accounts for up to 30% of propofol clearance, which explains the rapid clearance of propofol, which exceeds liver blood flow. The lungs also may play a role in the extrahepatic propofol metabolism. In sheep, the lungs are responsible for approximately 30% of the uptake and first-pass elimination after a bolus dose. In humans, a 20% to 30% decrease in propofol concentration measured across the lung exists with a higher concentration of the metabolite 2,6-diisopropyl 1,4-quinol on the arterial side of the circulation.
Propofol is generally known for its hemodynamic depressant effects and may reduce hepatic blood flow. As such, it may reduce the clearance of other drugs metabolized by the liver, in particular those with a high extraction ratio. In addition, propofol is known as a CYP3A4 inhibitor. In contrast to enzyme induction that may take several days or weeks to develop, competitive inhibition of CYP activity may occur almost instantaneously due to the competition of two drugs (e.g., propofol and midazolam) for the enzyme’s active site. A short-term exposure to propofol at a blood concentration of 3 μg/mL already reduces the CYP3A4 activity by about 37%.
Fospropofol is a water-soluble prodrug of propofol and is chemically described as phosphono- O -methyl-2, 6-diisopropylphenol, disodium salt (C 13 H 19 O 5 PNa 2 ). Fospropofol is metabolized by alkaline phosphatases to propofol, formaldehyde, and phosphate. Formaldehyde is further metabolized to formate which is then eliminated, primarily by oxidation to carbon dioxide. Over 71% of fospropofol is recovered in the urine within 192 hours following a single 400 mg IV dose. Renal elimination is <0.02% and total body clearance in the order of 0.28 L/h/kg. The terminal elimination half-life of fospropofol is 0.88 hours. The pharmacokinetics of fospropofol and liberated propofol are not affected by race, sex, or mild to moderate renal impairment. Furthermore, fospropofol pharmacokinetics are not affected by age or alkaline phosphatase concentration. So far, no pharmacokinetic interactions have been found between fospropofol and fentanyl, midazolam, morphine, or with propofol. This is probably because fospropofol is not subject to cytochrome P450 enzyme mediated metabolism.
The pharmacokinetics of propofol have been described by two-compartment and three-compartment models ( Table 23.1 ). [CR] After a single bolus dose, whole blood propofol levels decrease rapidly as a result of redistribution and elimination ( Fig. 23.2 ). The initial distribution half-life of propofol is 2 to 8 minutes. Studies in which the disposition of propofol is described by a three-compartment model give initial and slow distribution half-lives of 1 to 8 minutes and 30 to 70 minutes and an elimination half-life of 4 to 23.5 hours. The context-sensitive half-time for propofol ( Fig. 23.3 ) for infusions of up to 8 hours is less than 40 minutes. Because the required decrease in concentration for awakening after anesthesia or sedation with propofol is generally less than 50%, recovery from propofol remains rapid even after prolonged infusion. The volume of distribution of the central compartment has been calculated between 6 and 40 L, and the volume of distribution at steady state has been calculated as 150 to 700 L. The central compartment generally is smaller in the elderly as a result of the reduced cardiac output in these patients. A reduced cardiac output is associated with a higher peak plasma concentration, which is reflected by a smaller central compartment in the pharmacokinetic analysis. The clearance of propofol is extremely high—1.5 to 2.2 L/min. As discussed earlier, this exceeds hepatic blood flow, and extrahepatic metabolism has been shown.
Elimination | Elimination Half-Life (h) | Clearance (mL/kg/min) | Vd SS (L/kg) |
---|---|---|---|
Dexmedetomidine | 2-3 | 10-30 | 2-3 |
Diazepam | 20-50 | 0.2-0.5 | 0.7-1.7 |
Droperidol | 1.7-2.2 | 14 | 2 |
Etomidate | 2.9-5.3 | 18-25 | 2.5-4.5 |
Flumazenil | 0.7-1.3 | 5-20 | 0.6-1.6 |
Ketamine | 2.5-2.8 | 12-17 | 3.1 |
Lorazepam | 11-22 | 0.8-1.8 | 0.8-1.3 |
Methohexital | 2-6 | 10-15 | 1.5-3 |
Midazolam | 1.7-2.6 | 6.4-11 | 1.1-1.7 |
Propofol | 4-7 | 20-30 | 2-10 |
Thiopental | 7-17 | 3-4 | 1.5-3 |


The equilibrium constant for propofol based on suppression of the activity in the electroencephalogram (EEG) is about 0.3 min, and the half-life of equilibrium (T½ ke0 ) between plasma concentration and EEG effect is 2.5 minutes. The time to peak effect is 90 to 100 seconds. The onset of EEG effect with propofol seems to be independent of age. The onset of decreasing arterial blood pressure is much slower (double the time) and increases with age. For EEG and blood pressure changes, elderly patients show a concentration dependent increasing sensitivity. The pharmacokinetics of propofol may be altered by various factors (e.g., gender, weight, preexisting disease, age, and concomitant medication). Some studies suggest that propofol may exhibit nonlinear pharmacokinetics. Because propofol has a high extraction ratio, this may impair its own clearance by decreasing cardiac output and thus hepatic blood flow. As a result, a doubling of the dose of propofol may lead to a drug concentration that may be more than twice that initially experienced. In contrast, an increase in cardiac output induced by sympaticomimetic administration may lead to a decrease in the propofol plasma concentration. In a hemorrhagic shock model propofol concentrations increased 20% until uncompensated shock occurred, when there was a rapid and marked increase in propofol concentrations.
In term and preterm neonates, variability of propofol clearance was accounted for largely by postmenstrual and postnatal age with very fast maturation of clearance in neonatal life. Dosing in these neonates needs to be calculated with extreme care. Women have a larger volume of distribution and higher clearance rates, but the elimination half-life is similar for males and females. Elderly individuals have decreased clearance rates and a smaller central compartment volume. Both may be the result of a reduced cardiac output. Because of this and because of an increased sensitivity to the propofol effect in the elderly, patients aged 80 years or older generally need 50% of the propofol dose of patients aged 20 years old to target the same level of sedation or hypnosis. Children have a relatively larger central compartment volume (50%) and a more rapid clearance (25%). In children older than 3 years, volumes and clearances should be weight adjusted (also see Chapter 77 ). Children younger than 3 years of age also show weight-proportional pharmacokinetic parameters, but with larger central compartment and systemic clearance values than in adults or older children. This finding explains the larger dose requirements in this age group. Hepatic disease seems to result in larger steady state and central compartment volumes; clearance is unchanged, but the elimination half-life is slightly prolonged, as is time to recovery. In clinical practice, no significant dose adjustment is required in case of hepatic disease. The extrahepatic clearance of propofol that may compensate for a reduced hepatic function may be responsible for this.
Midazolam affects the pharmacokinetics of propofol. In the presence of a sedative midazolam concentration of 200 ng/mL, the blood propofol concentrations become elevated by about 25%. Midazolam reduces propofol metabolic clearance from 1.94 to 1.61 L/min, Cl 2 from 2.86 to 1.52 L/min, and Cl 3 from 0.95 to 0.73 L/min. The high extraction ratio of propofol of 0.79 to 0.92 suggests that the metabolic clearance of propofol may not be affected by enzyme inhibition but may be susceptible to changes in hepatic perfusion. The changes in the pharmacokinetics of propofol induced by midazolam thus may be the result of the hemodynamic alterations induced by the coadministration of midazolam.
Propofol in its turn affects midazolam pharmacokinetics as well. In the presence of sedative concentrations of propofol, plasma midazolam concentrations increased by 27%. In the presence of propofol, midazolam is administered in a smaller central compartment from which midazolam is cleared and distributed less rapidly to peripheral tissues. For example, alfentanil has been shown to increase blood propofol concentrations through a reduction in the elimination and distribution clearance of propofol. This is in line with other pharmacokinetic interactions between hypnotics and opioids when combined with propofol. Propofol has been shown to increase alfentanil concentrations by decreasing the elimination and the rapid and slow distribution clearances of alfentanil. Coadministration of propofol increased remifentanil concentrations via both a decrease in the central volume of distribution and distributional clearance of remifentanil by 41% and elimination clearance by 15%. Propofol kinetics is unaltered by renal disease.
As previously stated, pharmacokinetic data on the disposition of fospropofol are scarce. Phase I and phase II studies were conducted in Europe when a detection error became apparent resulting in the retraction of six published manuscripts. Currently, no further pharmacokinetic studies have been initiated. The pharmacokinetics of fospropofol in humans remains largely unknown.
Fospropofol protein binding is extensive (98%). It has a small volume of distribution of 0.3 L/kg, a total body clearance of 0.36 L/kg/h with a terminal elimination half-life of 0.88 hours. After a bolus dose of 6 mg/kg of fospropofol, the parent drug peaks at 4 minutes and is rapidly metabolized to propofol with a peak plasma propofol concentration at 12 minutes after administration of fospropofol. With this fospropofol dose the highest plasma concentration reached (Cmax) of fospropofol was 78.7 μg/mL and the Cmax of propofol was 1.08 μg/mL. The total body clearance of fospropofol and propofol were 0.36 and 3.2 L/kg/h. The terminal half-lives were 0.88 and 1.13 hours, respectively.
Pharmacodynamics
Effects on the Central Nervous System
The hypnotic action of propofol is mostly mediated by enhancing γ-aminobutyric acid (GABA)-induced chloride current through its binding to the β-subunit of GABA A receptor. Sites on the β 1 -subunit, β 2 -subunit, and β 3 -subunit of the transmembrane domains are crucial for the hypnotic action of propofol. The α-subunit and γ 2 -subunit subtypes also seem to contribute to modulating the effects of propofol on the GABA receptor. The effect of propofol has been described in two manners—indirect and direct. Propofol exhibits an indirect effect by potentiation of the ion channel activation by GABA, shifting the concentration-response relationship to the left. At higher propofol concentrations, propofol is also thought to directly activate GABA A receptor channels. The exact mechanism and location of changes that are associated with the change from consciousness to the unconscious state are not yet fully understood. Some experts suggest that a proper functioning of the brain stem-thalamo-cortical arousal circuits are critical while others state that consciousness is more related to fronto-parietal association cortex activity. Propofol, through its action on GABA A receptors in the hippocampus, inhibits acetylcholine release in the hippocampus and prefrontal cortex. The α 2 -adrenoreceptor system also seems to play an indirect role in the sedative effects of propofol. Resting state fMRI studies suggest that propofol’s action may be related to a CNS that reduces its discriminable state and switches into stereotypic patterns of firing under propofol sedation. The so called default mode network (DMN), including the posterior cingulated, the medial frontal cortex, and bilateral parietal cortices, is the anatomical substrate in which these stereotypical patterns become visible. Using positron emission tomography, propofol hypnosis is related with reduced activity in the thalamic and precuneus regions. These regions likely play an important role in propofol-induced unconsciousness.
Propofol results also in widespread inhibition of the N -methyl-d-aspartate (NMDA) subtype of glutamate receptor through modulation of sodium channel gating, an action that also may contribute to the drug’s CNS effects. Propofol has a direct depressant effect on neurons of the spinal cord. In acutely dissociated spinal dorsal horn neurons, propofol acts on GABA A and glycine receptors. The sense of well-being in patients with propofol is related to the increase in dopamine concentrations in the nucleus accumbens (a phenomenon noted with drugs of abuse and pleasure-seeking behavior). Propofol’s antiemetic action may be explained by the decrease in serotonin levels it produces in the area postrema, probably through its action on GABA receptors.
The onset of hypnosis after a dose of 2.5 mg/kg is rapid (one arm–brain circulation), with a peak effect seen at 90 to 100 seconds. The median effective dose (ED 50 ) of propofol for loss of consciousness is 1 to 1.5 mg/kg after a bolus. The duration of hypnosis is dose-dependent, being 5 to 10 minutes after 2 to 2.5 mg/kg. Age markedly affects the induction dose, which is largest at ages younger than 2 years (ED 95 2.88 mg/kg) and decreases with increasing age. This is a direct result of the altered pharmacokinetics in children and elderly. Children exhibit a relatively larger central compartment and therefore need a higher dose to assure a similar blood-drug concentration. In addition, the rapid clearance of propofol in children requires a larger maintenance dose as well. Increasing age decreases the propofol concentration required for loss of consciousness.
At subhypnotic doses, propofol provides sedation and amnesia. Propofol infusions of at least 2 mg/kg/h were necessary to provide amnesia in unstimulated volunteers. Awareness during surgery at higher infusion rates has been reported. During surgical procedures, extremely high infusion rates producing blood propofol concentrations in excess of 10 μg/mL may be necessary to prevent awareness if propofol is used as the sole anesthetic. Propofol also tends to produce a general state of well-being. Hallucinations, sexual fantasies, and opisthotonos occur after propofol administration.
The effect of propofol on the EEG, as assessed after 2.5 mg/kg followed by an infusion, shows an initial increase in alpha rhythm followed by a shift to gamma and theta frequency. Rapid infusion rates produce burst suppression at blood propofol concentrations higher than 8 μg/mL. Propofol causes a concentration-dependent decrease in the bispectral index (BIS), with 50% and 95% of patients unable to respond to a verbal command at a BIS of 63 and 51, respectively. The propofol concentration at which 50% of volunteers failed to respond to verbal command was 2.35 μg/mL. Lack of recall was observed in 95% of patients at a BIS value of 77. Propofol effect site concentrations provide similar correlation with decreases in the spectral entropy variable derived from the EEG as it does with BIS, and a similar ability to titrate propofol anesthetic effect. The effect of propofol on epileptogenic EEG activity is controversial. Propofol may suppress seizure activity via GABA agonism, inhibition of NMDA receptors, and modulation of slow calcium ion channels. However, the same GABA agonism and glycine antagonism may also induce clinical seizures and EEG epileptiform changes. This especially may occur during induction of and emergence from anesthesia. Propofol has a dose-dependent anticonvulsant. Propofol has even been used to treat epileptic seizures. Yet propofol can cause grand mal seizures and has been used for cortical mapping of epileptogenic foci.
Unfortunately, propofol can be addictive. An important issue in the potential of abuse is the development of tolerance. Tolerance to a drug creates circumstances for abuse. Propofol is being used as a sedative in the intensive care unit (ICU); in 20% to 40% of patients, the propofol dosage regimen needs to be repeatedly adjusted upward in order to maintain the same effect. Data on propofol abuse in the general public are unknown but the incidence of abuse is likely to be low compared to other substances. For healthcare workers, propofol is easy to access and case reports of lethal self-administration do occur. Some have suggested that there are more frequent incidences of propofol abuse by healthcare providers and support stricter propofol regulation. In contrast to propofol, fospropofol was classified in 2009 by the US Drug Enforcement Administration (DEA) as a controlled substance.
Propofol decreases intracranial pressure (ICP) in patients with either normal or increased ICP (also see Chapter 57 ). The decrease in ICP (30% to 50%) is associated with significant decreases in cerebral perfusion pressure (CPP). The use of propofol in head-injured patients should be restricted to doses providing mild-to-moderate sedation (i.e., blood concentration of 2 μg/mL, infusion of 25 to 75 μg/kg/min). Anesthetics are neuroprotective because they reduce the metabolic oxygen use that is beneficial for the balance between energy supply and demand and because they increase the tolerance to hypoxia by the neuronal tissue. Propofol has no direct preconditioning effect but may attenuate glutamate-mediated excitotoxicity. Propofol acutely reduces intraocular pressure by 30% to 40%. Compared with thiopental, propofol produces a larger decrease in intraocular pressure and is more effective in preventing an increase in intraocular pressure secondary to succinylcholine and endotracheal intubation. Normal cerebral reactivity to carbon dioxide and autoregulation are maintained during a propofol infusion.
The neuroprotective effects of propofol remain controversial. In an incomplete ischemia model in rats, propofol administered to burst suppression results in significantly better neurologic outcome and less brain tissue injury compared with fentanyl. Propofol administered at sedative concentrations started either immediately after or at 1 hour after an ischemic insult significantly reduced infarct size compared with awake controls infused with intralipid. Subanesthetic doses of propofol also induce neuroapoptosis in the infant mouse brain. In addition, anesthetic doses of propofol in rats induce complex changes that are accompanied by cell death in the cortex and thalamus of the developing rat brain. The neuronal protective effect of propofol may be due to the attenuation of changes in adenosine triphosphate, calcium, sodium, and potassium caused by hypoxic injury and its antioxidant action by inhibiting lipid peroxidation. Current evidence indicates that propofol can protect neurons against ischemic injury caused by excitotoxicity, but neuroprotection may be sustained only if the ischemic insult is relatively mild and is not sustained after a prolonged recovery period. Prolonged propofol sedation in children is associated with adverse neurologic sequelae.
Many anesthetic-related drugs decrease the required dose or blood concentrations of propofol’s pharmacologic action. The “required dose” is usually directly related to the required concentration for a given effect. The propofol Cp 50 (blood concentration needed for 50% of subjects to not respond to a defined stimulus) for loss of response to verbal command in the absence of any other drug is 2.3 to 3.5 μg/mL. The propofol Cp 50 to prevent movement on skin incision is 16 μg/mL; this is markedly reduced by increasing concentrations (i.e., doses) of fentanyl or alfentanil. The propofol Cp 50 for skin incision when combined with benzodiazepine premedication (lorazepam, 1 to 2 mg) and 66% nitrous oxide is 2.5 μg/mL (venous). This concentration is reduced to 1.7 μg/mL when morphine (0.15 mg/kg) rather than lorazepam is used for premedication. The concentration of propofol (when combined with 66% nitrous oxide) required during minor surgery is 1.5 to 4.5 μg/mL, and the concentration for major surgery is 2.5 to 6 μg/mL. Awakening usually occurs at concentrations less than 1.6 μg/mL and orientation occurs at concentrations less than 1.2 μg/mL when the propofol concentration is decreasing. Not surprisingly, awakening is postponed in the presence of high blood concentrations of opioids. Optimal propofol blood concentrations have been defined when combined with several opioids including remifentanil, alfentanil, sufentanil, and fentanyl that assure adequate anesthesia and the most rapid return to consciousness, postoperatively ( Table 23.2 ). In the presence of remifentanil, a relatively large-dose opioid anesthetic is recommended. Yet, with fentanyl an accompanying large dose of propofol should be used to assure rapid return to recovery postoperatively ( Fig. 23.4 ). When equilibration between blood and effect site is allowed, however, awakening concentrations (2.2 μg/mL) are similar to concentrations associated with loss of verbal command.
Opioid | Alfentanil EC50-EC95 (90-130 ng/mL) | Fentanyl EC50-EC95 (1.1-1.6 ng/mL) | Sufentanil EC50-EC95 (0.14-0.20 ng/mL) | Remifentanil EC50-EC95 (4.7-8.0 ng/mL) |
---|---|---|---|---|
Bolus | 25-35 μg/kg in 30 sec | 3 μg/kg in 30 sec | 0.15-0.25 μg/kg in 30 sec | 1.5-2 μg/kg in 30 sec |
Infusion 1 | 50-75 μg/kg/h for 30 min | 1.5-2.5 μg/kg/h for 30 min | 0.15-0.22 μg/kg thereafter | 13-22 μg/kg/h for 20 min |
Infusion 2 | 30-42.5 μg/kg/h thereafter | 1.3-2 μg/kg/h up to 150 min | 11.5-19 μg/kg/h thereafter | |
Infusion 3 | 0.7-1.4 μg/kg/h thereafter | |||
Propofol | Propofol EC 50 -EC 95 (3.2-4.4 μ g/mL) | Propofol EC 50 -EC 95 (3.4-5.4 μ g/mL) | Propofol EC 50 -EC 95 (3.3-4.5 μ g/mL) | Propofol EC 50 -EC 95 (2.5-2.8 μ g/mL) |
Bolus | 2.0-2.8 mg/kg in 30 s | 2.0-3.0 mg/kg in 30 s | 2.0-2.8 mg/kg in 30 s | 1.5 mg/kg in 30 s |
Infusion 1 | 9-12 mg/kg/h for 40 min | 9-15 mg/kg/h for 40 min | 9-12 mg/kg/h for 40 min | 7-8 mg/kg/h for 40 min |
Infusion 2 | 7-10 mg/kg/h for 150 min | 7-12 mg/kg/h for 150 min | 7-10 mg/kg/h for 150 min | 6-6.5 mg/kg/h for 150 min |
Infusion 3 | 6.5-8 mg/kg/h thereafter | 6.5-11 mg/kg/h thereafter | 6.5-8 mg/kg/h thereafter | 5-6 mg/kg/h thereafter |
∗ When given in combination, within +/− 15% of the effect-site concentrations that are associated with a 50% and 95% probability of no response to surgical stimuli and the most rapid possible return of consciousness after termination of the infusions.

Effects on the Respiratory System
Apnea occurs after administration of an induction dose of propofol; the incidence and duration of apnea depend on dose, speed of injection, and concomitant premedication. An induction dose of propofol results in a 25% to 30% incidence of apnea from the respiratory depressant effects of propofol and yet a normal partial pressure of carbon dioxide in the blood (PaCO 2) at induction in the absence of surgical stimulation. Metabolic depression further prevents the PaCO 2 to increase. Yet, the duration of apnea occurring with propofol may be prolonged to more than 30 seconds. The incidence of prolonged apnea (>30 seconds) is increased further by addition of an opiate, either as premedication or just before induction of anesthesia. A maintenance infusion of propofol (100 μg/kg/min) results in a 40% decrease in tidal volume and a 20% increase in respiratory frequency, with an unpredictable change in minute ventilation. Doubling the infusion rate from 100 to 200 μg/kg/min causes a further moderate decrease in tidal volume but no change in respiratory frequency. As with other hypnotic drugs, spontaneous ventilation is the result of the respiratory depressant effects of the hypnotic agents and the decrease in CO 2 production resulting from the metabolic depression versus the stimulatory effects of the increasing PaCO 2 resulting from apnea and the level of nociception. Propofol (50-120 μg/kg/min) also depresses the ventilatory response to hypoxia, presumably by a direct action on carotid body chemoreceptors. Propofol induces bronchodilation in patients with chronic obstructive pulmonary disease. Propofol attenuates vagal (at low concentrations) and methacholine-induced (at high concentrations) bronchoconstriction and seems to have a direct action on muscarinic receptors. Propofol inhibits the receptor-coupled signal transduction pathway through inositol phosphate generation and inhibition of Ca 2+ mobilization. The preservative used with propofol is important regarding its bronchodilator activity. Propofol with metabisulfite (compared with propofol without metabisulfite) does not inhibit vagal or methacholine-induced bronchoconstriction. Propofol potentiates hypoxic pulmonary vasoconstriction, an effect caused by inhibition of K(+) (ATP)-mediated pulmonary vasodilatation. Propofol has an impact on the pulmonary pathophysiology of adult respiratory distress syndrome. In an animal model of septic endotoxemia, propofol (10 mg/kg/h) significantly reduced free radical mediated and cyclooxygenase catalyzed lipid peroxidation. In addition, PaO 2 and hemodynamics were maintained closer to baseline. These benefits of propofol have not yet been confirmed in humans.
Effects on the Cardiovascular System
The cardiovascular effects of propofol for induction and maintenance of anesthesia have been evaluated ( Table 23.3 ). The most prominent effect of propofol is a decrease in arterial blood pressure during induction of anesthesia. [CR] Independent of the presence of cardiovascular disease, an induction dose of 2 to 2.5 mg/kg produces a 25% to 40% reduction of systolic blood pressure. Similar changes are seen in mean and diastolic blood pressure. The decrease in arterial blood pressure is associated with a decrease in cardiac output/cardiac index (±15%), stroke volume index (±20%), and systemic vascular resistance (15%-25%). Left ventricular stroke work index also is decreased (±30%). When looking specifically at right ventricular function, propofol produces a marked reduction in the slope of the right ventricular end-systolic pressure-volume relationship.
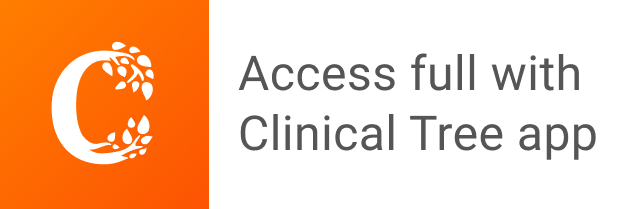