Key Points
- ▪
The introduction of thiopental into clinical practice in 1934 marked the beginning of modern intravenous (IV) anesthesia. Today, IV anesthetics are used for induction and maintenance of anesthesia and sedation in a wide variety of circumstances.
- ▪
The most commonly used IV anesthetic is propofol, an alkylphenol presently formulated in a lipid emulsion. Propofol provides rapid onset and offset with context-sensitive decrement times of approximately 10 minutes when infused for less than 3 hours and less than 40 minutes when infused for up to 8 hours. Its mechanism of action is likely the enhancement of gamma-aminobutyric acid (GABA)-induced chloride currents. Propofol causes a dose-dependent decrease in arterial blood pressure predominantly through a decrease in systemic vascular resistance and causes moderate respiratory depression. A unique action of propofol is its antiemetic effect, even at concentrations less than those producing sedation. A growing body of evidence suggests propofol may have antitumor potential.
- ▪
Barbiturates were the most commonly used IV drugs administered to induce anesthesia prior to the introduction of propofol. Thiopental provides rapid onset and offset when used as a single dose, but it accumulates rapidly with repeated or prolonged administration thus postponing recovery from anesthesia. Methohexital has a rapid onset and offset similar to propofol for procedures lasting less than 2 hours. The barbiturates are administered as sodium salts diluted in a water base at an alkaline pH. Similar to propofol, the barbiturates provide their hypnotic effects largely through action on the GABAA receptor. Barbiturates provide cerebral protection and are, apart from induction of anesthesia, used primarily for this purpose. They cause a moderate dose-dependent decrease in arterial blood pressure (primarily as a result of peripheral vasodilation) and respiratory drive. The barbiturates are contraindicated in patients with porphyria.
- ▪
The benzodiazepines are used primarily for anxiolysis and amnesia or for conscious sedation. The water-soluble benzodiazepine midazolam is most frequently used intravenously because of its rapid onset and offset compared with other benzodiazepines (e.g., diazepam). The onset time of midazolam is slower than that of propofol and barbiturates, and its offset, especially with larger doses or a prolonged infusion, is considerably longer than that of propofol or methohexital and may be prolonged in hepatic and renal failure. The benzodiazepines act through the GABA receptor. Flumazenil is a specific benzodiazepine antagonist. It can be used to reverse the effects of benzodiazepines but should be used with caution because its antagonizing effect often lasts shorter than the benzodiazepine effect that it is supposed to antagonize. The benzodiazepines generally produce only a mild decrease in arterial blood pressure and mild-to-moderate respiratory depression. Remimazolam is the most recent benzodiazepine with an ultrashort duration of action due to its rapid clearance through plasma esterases.
- ▪
Ketamine is a phencyclidine derivative that acts primarily, but not entirely, as antagonist of the N-methyl- d -aspartate (NMDA) receptor. It produces a dissociative state of hypnosis and analgesia. It has been used for induction and maintenance of anesthesia. Ketamine is associated with significant adverse psychological effects from larger doses and has several other side effects. It is used now primarily for its analgesic properties. It has rapid onset and relatively rapid offset, even after an infusion of several hours. It has sympathomimetic effects that preserve cardiac function. Ketamine has minimal effect on respiration and tends to preserve autonomic reflexes. In addition, ongoing research suggests ketamine may play a role as an antidepressant.
- ▪
Etomidate is an imidazole derivative used primarily for induction of anesthesia, especially in elderly patients and patients who are cardiovascularly compromised. It has a rapid onset of effect and a rapid offset even after a continuous infusion. A dose used to induce anesthesia results in inhibition of adrenocortical synthesis and possible mortality in intensive care unit (ICU) patients. The major advantage of etomidate is its minimal effect on the cardiovascular and respiratory systems.
- ▪
Dexmedetomidine is the most recently released IV anesthetic. It is a highly selective α 2 -adrenergic agonist that produces sedation, sympatholysis, hypnosis, and analgesia. Dexmedetomidine is approved for ICU sedation of initially intubated and mechanically ventilated patients, up to 24 hours. It may be advantageous for its ability to prevent delirium. Its second indication is procedural sedation of nonintubated patients. With increasing frequency dexmedetomine finds its use as a sedative during invasive or radiological procedures and as an adjunct in central or peripheral neural blockade. Its primary action is as an agonist on α 2 -receptors in the locus caeruleus. It has minimal effect on respiration. Heart rate and cardiac output show a concentration-dependent decrease.
- ▪
Droperidol, a butyrophenone and major tranquilizer, was initially used to produce a state of neuroleptanesthesia. Its prolongation of the QT interval has resulted in its withdrawal in several countries and its limitation to the treatment of postoperative nausea and vomiting (PONV) with a black box warning in the United States. Because the use of low-dose droperidol (<1.25 mg) for PONV has not been approved by the US Food and Drug Administration (FDA), the black box warning does not relate to this use. Clinically significant prolongation of the QT interval by doses used for PONV (0.625 to 1.25 mg) has been challenged by several editorials, and this effect has not been substantiated by review of the reported cases or other literature. Low-dose droperidol remains an effective antiemetic therapy and is used as such in many European countries (also see Chapter 80 ).
Acknowledgment
The editors and publisher would like to thank Drs. J.G. Reeves, Peter S.A. Glass, David A. Lubarsky, Matthew D. McEvoy, and Richardo Martinez-Ruiz for contributing a chapter on this topic in a previous edition of this work. It has served as the foundation for the current chapter.
Intravenous (IV) anesthesia can be traced back to 1656 with Percival Christopher Wren’s and Daniel Johann Major’s first experiments with the IV using a goose quill and bladder to inject wine and ale into a dog’s vein. In 1665 German naturalist and physician Sigismund Elsholz made the first attempt at IV anesthesia in humans and investigated the possibilities of IV injection with opiates. IV anesthesia further evolved when Fedoroff started using hedonal in St. Petersburg in 1905 and entered the era of modern anesthesia with the release of thiopental in 1936. Since these beginnings, and in particular during the past three decades, the pharmacokinetics and pharmacodynamics of IV anesthetics and their interactions have been described in increasingly greater detail. This body of knowledge and the availability of increasingly shorter-acting drugs now allow the anesthesia provider to administer anesthesia not on the basis of the needs of the population but to focus anesthesia on the individual needs of the patient. Today’s anesthesia provider is supported by modern IV drug administration techniques like target-controlled infusion and central nervous system (CNS) monitoring devices to further optimize and individualize the application of IV anesthesia. This chapter describes the current status of the pharmacology of IV anesthetics and their place in modern anesthesia.
Propofol
History
Since its introduction in the 1970s, propofol has become the most used IV hypnotic today. Building on work on the sedative properties of phenol derivates in mice, propofol was developed in the United Kingdom by Imperial Chemical Industries as ICI 35868. The initial solution of propofol, as released in 1977 in Cremophor EL, was withdrawn because of anaphylactic reactions, and replaced and reformulated as an emulsion of a soya oil/propofol mixture in water and relaunched in 1986. Propofol is used for induction and maintenance of anesthesia and for sedation in and outside the operating room.
Physicochemical Characteristics
Propofol ( Fig. 23.1 ) is one of a group of alkylphenols that were explored for their hypnotic properties in animals. The alkylphenols are highly lipid soluble and are insoluble in an aqueous solution. Numerous formulations of propofol are marketed today. The formulation most used is that of 1% propofol, 10% soybean oil, and 1.2% purified egg phospholipid added as emulsifier, with 2.25% of glycerol as a tonicity-adjusting agent, and sodium hydroxide to change the pH. Following concerns regarding microbial growth in the emulsion, EDTA was added for its bacteriostatic activities. Propofol has a pH of 7 and appears as a slightly viscous, milky white substance, a result of small lipid droplets in solution. In Europe, a 2% formulation and a formulation in which the emulsion contains a mixture of medium-chain and long-chain triglycerides also are available. All formulations commercially available are stable at room temperature, are not light sensitive, and may be diluted with 5% dextrose in water. Propofol concentrations may be measured both in whole blood and in the exhaled air.

In December 2008 the US Food and Drug Administration (FDA) approved fospropofol disodium (Lusedra) for monitored anesthesia care in adult patients undergoing diagnostic and therapeutic procedures. Fospropofol is a water-soluble prodrug of propofol that is metabolized by alkaline phosphatases in the liver to the active metabolite propofol. One millimole (mmol) of propofol is generated for each mmol of fospropofol sodium administered. About 1.86 mg of fospropofol sodium is the molar equivalent of 1 mg propofol. In April 2010, six studies on the pharmacokinetics and pharmacodynamics of fospropofol were retracted as a result of an analytical assay inaccuracy that was discovered after publication of these studies. Since then, few data on the pharmacokinetics and pharmacodynamics of fospropofol have been published. Although fospropofol remains available for monitored anesthesia care, data now available are scarce and most pharmacokinetic-pharmacodynamic data that are available come from the United States as described in a recent review. In contrast to propofol, fospropofol is not associated with pain on injection, although mild to moderate perineal paresthesias and pruritis minutes after a bolus injection of fospropofol have been reported and may be due to a phosphate metabolite.
Pharmacokinetics
Propofol is oxidized to 1,4-diisopropyl quinol in the liver. Propofol and 1,4-diisopropyl quinol are conjugated with glucuronic acid to propofol-1-glucuronide, quinol-1-glucuronide, and quinol-4-glucuronide, which then may be excreted by the kidneys. After a 2.5-hour anesthetic with propofol, patients excrete propofol and propofol metabolites for over 60 hours. Less than 1% propofol is excreted unchanged in urine, and only 2% is excreted in feces. The metabolites of propofol are thought to be inactive. Because clearance of propofol (>1.5 L/min) exceeds hepatic blood flow, extrahepatic metabolism or extrarenal elimination may occur. Extrahepatic metabolism has been confirmed during the anhepatic phase of patients receiving a transplanted liver with the determination of propofol metabolites after propofol administration in the absence of liver tissue. The most important extrahepatic site for propofol metabolism is the kidney. Renal metabolism of propofol accounts for up to 30% of propofol clearance, which explains the rapid clearance of propofol, which exceeds liver blood flow. The lungs also may play a role in the extrahepatic propofol metabolism. In sheep, the lungs are responsible for approximately 30% of the uptake and first-pass elimination after a bolus dose. In humans, a 20% to 30% decrease in propofol concentration measured across the lung exists with a higher concentration of the metabolite 2,6-diisopropyl 1,4-quinol on the arterial side of the circulation.
Propofol is generally known for its hemodynamic depressant effects and may reduce hepatic blood flow. As such, it may reduce the clearance of other drugs metabolized by the liver, in particular those with a high extraction ratio. In addition, propofol is known as a CYP3A4 inhibitor. In contrast to enzyme induction that may take several days or weeks to develop, competitive inhibition of CYP activity may occur almost instantaneously due to the competition of two drugs (e.g., propofol and midazolam) for the enzyme’s active site. A short-term exposure to propofol at a blood concentration of 3 μg/mL already reduces the CYP3A4 activity by about 37%.
Fospropofol is a water-soluble prodrug of propofol and is chemically described as phosphono- O -methyl-2, 6-diisopropylphenol, disodium salt (C 13 H 19 O 5 PNa 2 ). Fospropofol is metabolized by alkaline phosphatases to propofol, formaldehyde, and phosphate. Formaldehyde is further metabolized to formate which is then eliminated, primarily by oxidation to carbon dioxide. Over 71% of fospropofol is recovered in the urine within 192 hours following a single 400 mg IV dose. Renal elimination is <0.02% and total body clearance in the order of 0.28 L/h/kg. The terminal elimination half-life of fospropofol is 0.88 hours. The pharmacokinetics of fospropofol and liberated propofol are not affected by race, sex, or mild to moderate renal impairment. Furthermore, fospropofol pharmacokinetics are not affected by age or alkaline phosphatase concentration. So far, no pharmacokinetic interactions have been found between fospropofol and fentanyl, midazolam, morphine, or with propofol. This is probably because fospropofol is not subject to cytochrome P450 enzyme mediated metabolism.
The pharmacokinetics of propofol have been described by two-compartment and three-compartment models ( Table 23.1 ). [CR] After a single bolus dose, whole blood propofol levels decrease rapidly as a result of redistribution and elimination ( Fig. 23.2 ). The initial distribution half-life of propofol is 2 to 8 minutes. Studies in which the disposition of propofol is described by a three-compartment model give initial and slow distribution half-lives of 1 to 8 minutes and 30 to 70 minutes and an elimination half-life of 4 to 23.5 hours. The context-sensitive half-time for propofol ( Fig. 23.3 ) for infusions of up to 8 hours is less than 40 minutes. Because the required decrease in concentration for awakening after anesthesia or sedation with propofol is generally less than 50%, recovery from propofol remains rapid even after prolonged infusion. The volume of distribution of the central compartment has been calculated between 6 and 40 L, and the volume of distribution at steady state has been calculated as 150 to 700 L. The central compartment generally is smaller in the elderly as a result of the reduced cardiac output in these patients. A reduced cardiac output is associated with a higher peak plasma concentration, which is reflected by a smaller central compartment in the pharmacokinetic analysis. The clearance of propofol is extremely high—1.5 to 2.2 L/min. As discussed earlier, this exceeds hepatic blood flow, and extrahepatic metabolism has been shown.
Elimination | Elimination Half-Life (h) | Clearance (mL/kg/min) | Vd SS (L/kg) |
---|---|---|---|
Dexmedetomidine | 2-3 | 10-30 | 2-3 |
Diazepam | 20-50 | 0.2-0.5 | 0.7-1.7 |
Droperidol | 1.7-2.2 | 14 | 2 |
Etomidate | 2.9-5.3 | 18-25 | 2.5-4.5 |
Flumazenil | 0.7-1.3 | 5-20 | 0.6-1.6 |
Ketamine | 2.5-2.8 | 12-17 | 3.1 |
Lorazepam | 11-22 | 0.8-1.8 | 0.8-1.3 |
Methohexital | 2-6 | 10-15 | 1.5-3 |
Midazolam | 1.7-2.6 | 6.4-11 | 1.1-1.7 |
Propofol | 4-7 | 20-30 | 2-10 |
Thiopental | 7-17 | 3-4 | 1.5-3 |


The equilibrium constant for propofol based on suppression of the activity in the electroencephalogram (EEG) is about 0.3 min, and the half-life of equilibrium (T½ ke0 ) between plasma concentration and EEG effect is 2.5 minutes. The time to peak effect is 90 to 100 seconds. The onset of EEG effect with propofol seems to be independent of age. The onset of decreasing arterial blood pressure is much slower (double the time) and increases with age. For EEG and blood pressure changes, elderly patients show a concentration dependent increasing sensitivity. The pharmacokinetics of propofol may be altered by various factors (e.g., gender, weight, preexisting disease, age, and concomitant medication). Some studies suggest that propofol may exhibit nonlinear pharmacokinetics. Because propofol has a high extraction ratio, this may impair its own clearance by decreasing cardiac output and thus hepatic blood flow. As a result, a doubling of the dose of propofol may lead to a drug concentration that may be more than twice that initially experienced. In contrast, an increase in cardiac output induced by sympaticomimetic administration may lead to a decrease in the propofol plasma concentration. In a hemorrhagic shock model propofol concentrations increased 20% until uncompensated shock occurred, when there was a rapid and marked increase in propofol concentrations.
In term and preterm neonates, variability of propofol clearance was accounted for largely by postmenstrual and postnatal age with very fast maturation of clearance in neonatal life. Dosing in these neonates needs to be calculated with extreme care. Women have a larger volume of distribution and higher clearance rates, but the elimination half-life is similar for males and females. Elderly individuals have decreased clearance rates and a smaller central compartment volume. Both may be the result of a reduced cardiac output. Because of this and because of an increased sensitivity to the propofol effect in the elderly, patients aged 80 years or older generally need 50% of the propofol dose of patients aged 20 years old to target the same level of sedation or hypnosis. Children have a relatively larger central compartment volume (50%) and a more rapid clearance (25%). In children older than 3 years, volumes and clearances should be weight adjusted (also see Chapter 77 ). Children younger than 3 years of age also show weight-proportional pharmacokinetic parameters, but with larger central compartment and systemic clearance values than in adults or older children. This finding explains the larger dose requirements in this age group. Hepatic disease seems to result in larger steady state and central compartment volumes; clearance is unchanged, but the elimination half-life is slightly prolonged, as is time to recovery. In clinical practice, no significant dose adjustment is required in case of hepatic disease. The extrahepatic clearance of propofol that may compensate for a reduced hepatic function may be responsible for this.
Midazolam affects the pharmacokinetics of propofol. In the presence of a sedative midazolam concentration of 200 ng/mL, the blood propofol concentrations become elevated by about 25%. Midazolam reduces propofol metabolic clearance from 1.94 to 1.61 L/min, Cl 2 from 2.86 to 1.52 L/min, and Cl 3 from 0.95 to 0.73 L/min. The high extraction ratio of propofol of 0.79 to 0.92 suggests that the metabolic clearance of propofol may not be affected by enzyme inhibition but may be susceptible to changes in hepatic perfusion. The changes in the pharmacokinetics of propofol induced by midazolam thus may be the result of the hemodynamic alterations induced by the coadministration of midazolam.
Propofol in its turn affects midazolam pharmacokinetics as well. In the presence of sedative concentrations of propofol, plasma midazolam concentrations increased by 27%. In the presence of propofol, midazolam is administered in a smaller central compartment from which midazolam is cleared and distributed less rapidly to peripheral tissues. For example, alfentanil has been shown to increase blood propofol concentrations through a reduction in the elimination and distribution clearance of propofol. This is in line with other pharmacokinetic interactions between hypnotics and opioids when combined with propofol. Propofol has been shown to increase alfentanil concentrations by decreasing the elimination and the rapid and slow distribution clearances of alfentanil. Coadministration of propofol increased remifentanil concentrations via both a decrease in the central volume of distribution and distributional clearance of remifentanil by 41% and elimination clearance by 15%. Propofol kinetics is unaltered by renal disease.
As previously stated, pharmacokinetic data on the disposition of fospropofol are scarce. Phase I and phase II studies were conducted in Europe when a detection error became apparent resulting in the retraction of six published manuscripts. Currently, no further pharmacokinetic studies have been initiated. The pharmacokinetics of fospropofol in humans remains largely unknown.
Fospropofol protein binding is extensive (98%). It has a small volume of distribution of 0.3 L/kg, a total body clearance of 0.36 L/kg/h with a terminal elimination half-life of 0.88 hours. After a bolus dose of 6 mg/kg of fospropofol, the parent drug peaks at 4 minutes and is rapidly metabolized to propofol with a peak plasma propofol concentration at 12 minutes after administration of fospropofol. With this fospropofol dose the highest plasma concentration reached (Cmax) of fospropofol was 78.7 μg/mL and the Cmax of propofol was 1.08 μg/mL. The total body clearance of fospropofol and propofol were 0.36 and 3.2 L/kg/h. The terminal half-lives were 0.88 and 1.13 hours, respectively.
Pharmacodynamics
Effects on the Central Nervous System
The hypnotic action of propofol is mostly mediated by enhancing γ-aminobutyric acid (GABA)-induced chloride current through its binding to the β-subunit of GABA A receptor. Sites on the β 1 -subunit, β 2 -subunit, and β 3 -subunit of the transmembrane domains are crucial for the hypnotic action of propofol. The α-subunit and γ 2 -subunit subtypes also seem to contribute to modulating the effects of propofol on the GABA receptor. The effect of propofol has been described in two manners—indirect and direct. Propofol exhibits an indirect effect by potentiation of the ion channel activation by GABA, shifting the concentration-response relationship to the left. At higher propofol concentrations, propofol is also thought to directly activate GABA A receptor channels. The exact mechanism and location of changes that are associated with the change from consciousness to the unconscious state are not yet fully understood. Some experts suggest that a proper functioning of the brain stem-thalamo-cortical arousal circuits are critical while others state that consciousness is more related to fronto-parietal association cortex activity. Propofol, through its action on GABA A receptors in the hippocampus, inhibits acetylcholine release in the hippocampus and prefrontal cortex. The α 2 -adrenoreceptor system also seems to play an indirect role in the sedative effects of propofol. Resting state fMRI studies suggest that propofol’s action may be related to a CNS that reduces its discriminable state and switches into stereotypic patterns of firing under propofol sedation. The so called default mode network (DMN), including the posterior cingulated, the medial frontal cortex, and bilateral parietal cortices, is the anatomical substrate in which these stereotypical patterns become visible. Using positron emission tomography, propofol hypnosis is related with reduced activity in the thalamic and precuneus regions. These regions likely play an important role in propofol-induced unconsciousness.
Propofol results also in widespread inhibition of the N -methyl-d-aspartate (NMDA) subtype of glutamate receptor through modulation of sodium channel gating, an action that also may contribute to the drug’s CNS effects. Propofol has a direct depressant effect on neurons of the spinal cord. In acutely dissociated spinal dorsal horn neurons, propofol acts on GABA A and glycine receptors. The sense of well-being in patients with propofol is related to the increase in dopamine concentrations in the nucleus accumbens (a phenomenon noted with drugs of abuse and pleasure-seeking behavior). Propofol’s antiemetic action may be explained by the decrease in serotonin levels it produces in the area postrema, probably through its action on GABA receptors.
The onset of hypnosis after a dose of 2.5 mg/kg is rapid (one arm–brain circulation), with a peak effect seen at 90 to 100 seconds. The median effective dose (ED 50 ) of propofol for loss of consciousness is 1 to 1.5 mg/kg after a bolus. The duration of hypnosis is dose-dependent, being 5 to 10 minutes after 2 to 2.5 mg/kg. Age markedly affects the induction dose, which is largest at ages younger than 2 years (ED 95 2.88 mg/kg) and decreases with increasing age. This is a direct result of the altered pharmacokinetics in children and elderly. Children exhibit a relatively larger central compartment and therefore need a higher dose to assure a similar blood-drug concentration. In addition, the rapid clearance of propofol in children requires a larger maintenance dose as well. Increasing age decreases the propofol concentration required for loss of consciousness.
At subhypnotic doses, propofol provides sedation and amnesia. Propofol infusions of at least 2 mg/kg/h were necessary to provide amnesia in unstimulated volunteers. Awareness during surgery at higher infusion rates has been reported. During surgical procedures, extremely high infusion rates producing blood propofol concentrations in excess of 10 μg/mL may be necessary to prevent awareness if propofol is used as the sole anesthetic. Propofol also tends to produce a general state of well-being. Hallucinations, sexual fantasies, and opisthotonos occur after propofol administration.
The effect of propofol on the EEG, as assessed after 2.5 mg/kg followed by an infusion, shows an initial increase in alpha rhythm followed by a shift to gamma and theta frequency. Rapid infusion rates produce burst suppression at blood propofol concentrations higher than 8 μg/mL. Propofol causes a concentration-dependent decrease in the bispectral index (BIS), with 50% and 95% of patients unable to respond to a verbal command at a BIS of 63 and 51, respectively. The propofol concentration at which 50% of volunteers failed to respond to verbal command was 2.35 μg/mL. Lack of recall was observed in 95% of patients at a BIS value of 77. Propofol effect site concentrations provide similar correlation with decreases in the spectral entropy variable derived from the EEG as it does with BIS, and a similar ability to titrate propofol anesthetic effect. The effect of propofol on epileptogenic EEG activity is controversial. Propofol may suppress seizure activity via GABA agonism, inhibition of NMDA receptors, and modulation of slow calcium ion channels. However, the same GABA agonism and glycine antagonism may also induce clinical seizures and EEG epileptiform changes. This especially may occur during induction of and emergence from anesthesia. Propofol has a dose-dependent anticonvulsant. Propofol has even been used to treat epileptic seizures. Yet propofol can cause grand mal seizures and has been used for cortical mapping of epileptogenic foci.
Unfortunately, propofol can be addictive. An important issue in the potential of abuse is the development of tolerance. Tolerance to a drug creates circumstances for abuse. Propofol is being used as a sedative in the intensive care unit (ICU); in 20% to 40% of patients, the propofol dosage regimen needs to be repeatedly adjusted upward in order to maintain the same effect. Data on propofol abuse in the general public are unknown but the incidence of abuse is likely to be low compared to other substances. For healthcare workers, propofol is easy to access and case reports of lethal self-administration do occur. Some have suggested that there are more frequent incidences of propofol abuse by healthcare providers and support stricter propofol regulation. In contrast to propofol, fospropofol was classified in 2009 by the US Drug Enforcement Administration (DEA) as a controlled substance.
Propofol decreases intracranial pressure (ICP) in patients with either normal or increased ICP (also see Chapter 57 ). The decrease in ICP (30% to 50%) is associated with significant decreases in cerebral perfusion pressure (CPP). The use of propofol in head-injured patients should be restricted to doses providing mild-to-moderate sedation (i.e., blood concentration of 2 μg/mL, infusion of 25 to 75 μg/kg/min). Anesthetics are neuroprotective because they reduce the metabolic oxygen use that is beneficial for the balance between energy supply and demand and because they increase the tolerance to hypoxia by the neuronal tissue. Propofol has no direct preconditioning effect but may attenuate glutamate-mediated excitotoxicity. Propofol acutely reduces intraocular pressure by 30% to 40%. Compared with thiopental, propofol produces a larger decrease in intraocular pressure and is more effective in preventing an increase in intraocular pressure secondary to succinylcholine and endotracheal intubation. Normal cerebral reactivity to carbon dioxide and autoregulation are maintained during a propofol infusion.
The neuroprotective effects of propofol remain controversial. In an incomplete ischemia model in rats, propofol administered to burst suppression results in significantly better neurologic outcome and less brain tissue injury compared with fentanyl. Propofol administered at sedative concentrations started either immediately after or at 1 hour after an ischemic insult significantly reduced infarct size compared with awake controls infused with intralipid. Subanesthetic doses of propofol also induce neuroapoptosis in the infant mouse brain. In addition, anesthetic doses of propofol in rats induce complex changes that are accompanied by cell death in the cortex and thalamus of the developing rat brain. The neuronal protective effect of propofol may be due to the attenuation of changes in adenosine triphosphate, calcium, sodium, and potassium caused by hypoxic injury and its antioxidant action by inhibiting lipid peroxidation. Current evidence indicates that propofol can protect neurons against ischemic injury caused by excitotoxicity, but neuroprotection may be sustained only if the ischemic insult is relatively mild and is not sustained after a prolonged recovery period. Prolonged propofol sedation in children is associated with adverse neurologic sequelae.
Many anesthetic-related drugs decrease the required dose or blood concentrations of propofol’s pharmacologic action. The “required dose” is usually directly related to the required concentration for a given effect. The propofol Cp 50 (blood concentration needed for 50% of subjects to not respond to a defined stimulus) for loss of response to verbal command in the absence of any other drug is 2.3 to 3.5 μg/mL. The propofol Cp 50 to prevent movement on skin incision is 16 μg/mL; this is markedly reduced by increasing concentrations (i.e., doses) of fentanyl or alfentanil. The propofol Cp 50 for skin incision when combined with benzodiazepine premedication (lorazepam, 1 to 2 mg) and 66% nitrous oxide is 2.5 μg/mL (venous). This concentration is reduced to 1.7 μg/mL when morphine (0.15 mg/kg) rather than lorazepam is used for premedication. The concentration of propofol (when combined with 66% nitrous oxide) required during minor surgery is 1.5 to 4.5 μg/mL, and the concentration for major surgery is 2.5 to 6 μg/mL. Awakening usually occurs at concentrations less than 1.6 μg/mL and orientation occurs at concentrations less than 1.2 μg/mL when the propofol concentration is decreasing. Not surprisingly, awakening is postponed in the presence of high blood concentrations of opioids. Optimal propofol blood concentrations have been defined when combined with several opioids including remifentanil, alfentanil, sufentanil, and fentanyl that assure adequate anesthesia and the most rapid return to consciousness, postoperatively ( Table 23.2 ). In the presence of remifentanil, a relatively large-dose opioid anesthetic is recommended. Yet, with fentanyl an accompanying large dose of propofol should be used to assure rapid return to recovery postoperatively ( Fig. 23.4 ). When equilibration between blood and effect site is allowed, however, awakening concentrations (2.2 μg/mL) are similar to concentrations associated with loss of verbal command.
Opioid | Alfentanil EC50-EC95 (90-130 ng/mL) | Fentanyl EC50-EC95 (1.1-1.6 ng/mL) | Sufentanil EC50-EC95 (0.14-0.20 ng/mL) | Remifentanil EC50-EC95 (4.7-8.0 ng/mL) |
---|---|---|---|---|
Bolus | 25-35 μg/kg in 30 sec | 3 μg/kg in 30 sec | 0.15-0.25 μg/kg in 30 sec | 1.5-2 μg/kg in 30 sec |
Infusion 1 | 50-75 μg/kg/h for 30 min | 1.5-2.5 μg/kg/h for 30 min | 0.15-0.22 μg/kg thereafter | 13-22 μg/kg/h for 20 min |
Infusion 2 | 30-42.5 μg/kg/h thereafter | 1.3-2 μg/kg/h up to 150 min | 11.5-19 μg/kg/h thereafter | |
Infusion 3 | 0.7-1.4 μg/kg/h thereafter | |||
Propofol | Propofol EC 50 -EC 95 (3.2-4.4 μ g/mL) | Propofol EC 50 -EC 95 (3.4-5.4 μ g/mL) | Propofol EC 50 -EC 95 (3.3-4.5 μ g/mL) | Propofol EC 50 -EC 95 (2.5-2.8 μ g/mL) |
Bolus | 2.0-2.8 mg/kg in 30 s | 2.0-3.0 mg/kg in 30 s | 2.0-2.8 mg/kg in 30 s | 1.5 mg/kg in 30 s |
Infusion 1 | 9-12 mg/kg/h for 40 min | 9-15 mg/kg/h for 40 min | 9-12 mg/kg/h for 40 min | 7-8 mg/kg/h for 40 min |
Infusion 2 | 7-10 mg/kg/h for 150 min | 7-12 mg/kg/h for 150 min | 7-10 mg/kg/h for 150 min | 6-6.5 mg/kg/h for 150 min |
Infusion 3 | 6.5-8 mg/kg/h thereafter | 6.5-11 mg/kg/h thereafter | 6.5-8 mg/kg/h thereafter | 5-6 mg/kg/h thereafter |
∗ When given in combination, within +/− 15% of the effect-site concentrations that are associated with a 50% and 95% probability of no response to surgical stimuli and the most rapid possible return of consciousness after termination of the infusions.

Effects on the Respiratory System
Apnea occurs after administration of an induction dose of propofol; the incidence and duration of apnea depend on dose, speed of injection, and concomitant premedication. An induction dose of propofol results in a 25% to 30% incidence of apnea from the respiratory depressant effects of propofol and yet a normal partial pressure of carbon dioxide in the blood (PaCO 2) at induction in the absence of surgical stimulation. Metabolic depression further prevents the PaCO 2 to increase. Yet, the duration of apnea occurring with propofol may be prolonged to more than 30 seconds. The incidence of prolonged apnea (>30 seconds) is increased further by addition of an opiate, either as premedication or just before induction of anesthesia. A maintenance infusion of propofol (100 μg/kg/min) results in a 40% decrease in tidal volume and a 20% increase in respiratory frequency, with an unpredictable change in minute ventilation. Doubling the infusion rate from 100 to 200 μg/kg/min causes a further moderate decrease in tidal volume but no change in respiratory frequency. As with other hypnotic drugs, spontaneous ventilation is the result of the respiratory depressant effects of the hypnotic agents and the decrease in CO 2 production resulting from the metabolic depression versus the stimulatory effects of the increasing PaCO 2 resulting from apnea and the level of nociception. Propofol (50-120 μg/kg/min) also depresses the ventilatory response to hypoxia, presumably by a direct action on carotid body chemoreceptors. Propofol induces bronchodilation in patients with chronic obstructive pulmonary disease. Propofol attenuates vagal (at low concentrations) and methacholine-induced (at high concentrations) bronchoconstriction and seems to have a direct action on muscarinic receptors. Propofol inhibits the receptor-coupled signal transduction pathway through inositol phosphate generation and inhibition of Ca 2+ mobilization. The preservative used with propofol is important regarding its bronchodilator activity. Propofol with metabisulfite (compared with propofol without metabisulfite) does not inhibit vagal or methacholine-induced bronchoconstriction. Propofol potentiates hypoxic pulmonary vasoconstriction, an effect caused by inhibition of K(+) (ATP)-mediated pulmonary vasodilatation. Propofol has an impact on the pulmonary pathophysiology of adult respiratory distress syndrome. In an animal model of septic endotoxemia, propofol (10 mg/kg/h) significantly reduced free radical mediated and cyclooxygenase catalyzed lipid peroxidation. In addition, PaO 2 and hemodynamics were maintained closer to baseline. These benefits of propofol have not yet been confirmed in humans.
Effects on the Cardiovascular System
The cardiovascular effects of propofol for induction and maintenance of anesthesia have been evaluated ( Table 23.3 ). The most prominent effect of propofol is a decrease in arterial blood pressure during induction of anesthesia. [CR] Independent of the presence of cardiovascular disease, an induction dose of 2 to 2.5 mg/kg produces a 25% to 40% reduction of systolic blood pressure. Similar changes are seen in mean and diastolic blood pressure. The decrease in arterial blood pressure is associated with a decrease in cardiac output/cardiac index (±15%), stroke volume index (±20%), and systemic vascular resistance (15%-25%). Left ventricular stroke work index also is decreased (±30%). When looking specifically at right ventricular function, propofol produces a marked reduction in the slope of the right ventricular end-systolic pressure-volume relationship.
Diazepam | Droperidol | Etomidate ∗ | Ketamine | Lorazepam | Midazolam | Propofol | |
---|---|---|---|---|---|---|---|
HR | −9 ± 13 | Unchanged | −5 ± 10 | 0-59 | Unchanged | −14 ± 12 | −10 ± 10 |
MBP | 0-19 | 0-10 | 0-17 | 0 ± 40 | −7 to 20 | −12 to 26 | −10 to 40 |
SVR | −22 ± 13 | −5 to 15 | −10 ± 14 | 0 ± 33 | −10 to 35 | 0-20 | −15 to 25 |
PAP | 0-10 | Unchanged | −9 ± 8 | +44 ± 47 | — | Unchanged | 0-10 |
PVR | 0-19 | Unchanged | −18 ± 6 | 0 ± 33 | Unchanged | Unchanged | 0-10 |
PAO | Unchanged | +25 ± 50 | Unchanged | Unchanged | — | 0-25 | Unchanged |
RAP | Unchanged | Unchanged | Unchanged | +15 ± 33 | Unchanged | Unchanged | 0-10 |
CI | Unchanged | Unchanged | −20 ± 14 | 0 ± 42 | 0 ± 16 | 0-25 | −10 to 30 |
SV | 0-8 | 0-10 | 0-20 | 0-21 | Unchanged | 0-18 | −10 to 25 |
LVSWI | 0-36 | Unchanged | 0-33 | 0 ± 27 | — | −28 to 42 | −10 to 20 |
dP/dt | Unchanged | — | 0-18 | Unchanged | — | 0-12 | Decreased |
∗ The larger deviations are in patients with valvular disease.
In patients with valvular heart disease, pulmonary artery and pulmonary capillary wedge pressure also are reduced, a finding that implies the resultant decrease in pressure is due to a decrease in preload and afterload. Although the decrease in systemic pressure after an induction dose of propofol is due to vasodilation , the direct myocardial depressant effects of propofol are more controversial. The decrease in cardiac output after propofol administration may be via its action on sympathetic drive to the heart. The hemodynamic response to propofol lags significantly behind that of the hypnotic effect. The effect-site equilibration half-life of propofol is in the order of 2 to 3 minutes for the hypnotic effect and about 7 minutes for the hemodynamic depressant effect. This implies that hemodynamic depression increases in a few minutes after a patient has lost consciousness from an induction of anesthesia.
High concentrations of propofol abolish the inotropic effect of α- but not β-adrenoreceptor stimulation and enhance the lusitropic (relaxation) effect of β stimulation. Clinically, the myocardial depressant effect and the vasodilation are dose-dependent and plasma concentration-dependent. Propofol is a vasodilator due to a reduction in sympathetic activity. The mechanism of this activity is a combination of a direct effect on intracellular smooth muscle calcium mobilization, inhibition of prostacyclin synthesis in endothelial cells, reduction in angiotensin II–elicited calcium entry, activation of K adenosine triphosphate channels, and stimulation of nitric oxide. The stimulation of nitric oxide may be modulated by any intralipid rather than propofol itself.
Heart rate does not change significantly after an induction dose of propofol. Propofol either may reset or may inhibit the baroreflex, reducing the tachycardic response to hypotension. Propofol also decreases cardiac parasympathetic tone in a dose-dependent manner. Propofol has a minimal direct effect on sinoatrial node function or on normal atrioventricular and accessory pathway conduction. Propofol attenuates the heart rate response to atropine in a dose-dependent manner. Propofol suppresses atrial (supraventricular) tachycardias and probably should be avoided during electrophysiologic studies. The peak plasma concentrations obtained after a bolus dose are substantially higher than the concentrations seen with a continuous infusion and may reach concentrations up to 80 to 100 μg/mL. Because the vasodilatory and myocardial depressant effects are concentration-dependent, the decrease in arterial blood pressure from propofol during the infusion phase (maintenance of anesthesia) is much less than that seen after induction of anesthesia by an intravenous bolus administration of propofol. An infusion of propofol reduces myocardial blood flow and oxygen consumption. Thus, global myocardial oxygen supply-to-demand ratio is likely preserved. The cardioprotective effect of propofol versus volatile anesthetics in patients having cardiac surgery on- or off-cardiopulmonary bypass is less debatable. In two large studies comparing propofol with sevoflurane in patients undergoing cardiac surgery, postoperative troponin levels were lower and hemodynamic function better in patients receiving sevoflurane. A study comparing desflurane with propofol in patients undergoing off-pump coronary artery bypass showed similar results. In contrast, administration of a large-dose of propofol (120 μg/kg/min) or small-dose of propofol (60 μg/kg/min) during cardiopulmonary bypass, or titrating isoflurane throughout surgery, showed improved troponin levels and better hemodynamic function in the large-dose propofol group compared to the isoflurane or small-dose propofol group. This study suggests that cardio protection with propofol is dose-dependent. Lastly, combinations of propofol with inhaled anesthetics may offer an optimal pre- and postconditioning strategy in patients scheduled for coronary bypass surgery. Isoflurane preconditioning combined with propofol postconditioning acts synergistically in attenuating postischemic myocardial reperfusion injury as determined by surrogate markers of myocardial injury and function. Heart rate changes are variable when anesthesia is maintained with propofol. The extent of hypotension, the ability for the patient to compensate, and the use of any other concomitant drugs are likely the most important factors in determining what happens to the heart rate after propofol administration.
Other Effects
Propofol, similar to thiopental, does not enhance neuromuscular blockade produced by neuromuscular blocking drugs. Propofol produces no effect on the evoked electromyogram or twitch tension; however, good or acceptable tracheal intubating conditions after propofol alone have been reported. Propofol does not trigger malignant hyperthermia and is an appropriate choice in patients with this condition. Propofol after a single dose or a prolonged infusion does not affect corticosteroid synthesis or alter the normal response to adrenocorticotropic hormone (ACTH) stimulation. Propofol in the emulsion formulation does not alter hepatic, hematologic, or fibrinolytic function. Lipid emulsion per se reduces in vitro platelet aggregation, however. Anaphylactoid reactions to the present formulation of propofol have been reported. In at least some patients, the immune response was entirely due to propofol and not to the lipid emulsion. Most patients developing the anaphylactoid response to propofol had a previous history of allergic responses. Perhaps propofol should not be used in patients with multiple drug allergies. Propofol alone in intralipid does not trigger histamine release. Fospropofol is metabolized to propofol and formate. Formate concentrations do not increase after fospropofol administration. Propofol also possesses significant antiemetic activity with small (subhypnotic) doses (i.e., 10 mg in adults). The median concentration of propofol with an antiemetic effect was 343 ng/mL, which also causes a mild sedative effect. This concentration can be achieved by an initial dose of propofol infusion of 10 to 20 mg followed by 10 μg/kg/min. Propofol used as a maintenance anesthetic during breast surgery was more effective than 4 mg of ondansetron given as prophylaxis in preventing postoperative nausea and vomiting (PONV) (also see Chapter 80 ). Propofol as an infusion of 1 mg/kg/h (17 μg/kg/min) also has provided excellent antiemetic action after anticancer chemotherapy. At subhypnotic doses, propofol relieves cholestatic pruritus and is likely as effective as naloxone in treating pruritus induced by spinal opiates.
Propofol decreases polymorphonuclear leukocyte chemotaxis, but not adherence phagocytosis and killing. This action contrasts with the effect of thiopental, which inhibits all these chemotactic responses. However, propofol inhibits phagocytosis and killing of Staphylococcus aureus and Escherichia coli . There have been life-threatening systemic infections associated with the use of propofol. In hospitals where these infections occurred, opened vials and syringes of propofol had positive cultures. The intralipid that acts as the solvent for propofol is an excellent culture medium. Disodium edetate or metabisulfite has been added to the formulation of propofol in an attempt to retard such bacterial growth. Strict aseptic technique still must be observed. The administration of propofol is associated with the development of pancreatitis, which may be related to hypertriglyceridemia. Patients who developed hypertriglyceridemia tended to be older, had a longer ICU stay, and received propofol for a longer duration. If propofol is being used for prolonged sedation or at higher infusion rates (especially in elderly patients), serum triglyceride concentrations should be routinely monitored.
Uses
Induction and Maintenance of Anesthesia
Propofol is suitable for the induction and maintenance of anesthesia ( Box 23.1 ). The intravenous induction dose is 1 to 2.5 mg/kg. Physiologic characteristics that best determine the appropriate dose to induce anesthesia are age, lean body mass, and central blood volume. Propofol may be titrated on the basis of the BIS value for maintenance of anesthesia and to assure adequacy of anesthesia and prevention of overdosing. Premedication with an opiate or a benzodiazepine, or both, markedly reduces the necessary induction dose. The induction dose needs to be reduced in elderly patients and a dose of 1 mg/kg (with premedication) to 1.75 mg/kg (without premedication) is recommended for inducing anesthesia in patients older than 60. Furthermore, older and sicker (ASA class III to IV) patients develop more profound hypotension, especially when propofol islcombined with an opiate (also see Chapter 65 ). To prevent hypotension in sicker patients or in patients presenting for cardiac surgery, intravenously administered fluids should be given as tolerated, and propofol titrated to achieve the desired anesthetic state. In general, for both pharmacokinetic and pharmacodynamic reasons elderly patients (>80 years old) require half the dose of young patients (<20 years) . For induction in children, the ED 95 (2-3 mg/kg) is increased, primarily because of pharmacokinetic differences. Children demonstrate a smaller central compartment, an increased metabolic clearance, and larger volumes of distribution of propofol relative to adult patients. Propofol, when used for induction of anesthesia in short-lasting procedures, results in a significantly quicker recovery and an earlier return of psychomotor function compared with thiopental or methohexital, regardless of the anesthetic used for maintenance of anesthesia.
Induction of general anesthesia | 1-2.5 mg/kg IV, dose reduced with increasing age |
Maintenance of general anesthesia | 50-150 μg/kg/min IV combined with N 2 O or an opiate |
Sedation | 25-75 μg/kg/min IV |
Antiemetic action | 10-20 mg IV, can repeat every 5-10 min or start infusion of 10 μg/kg/min |
Several infusion schemes have been used to achieve adequate plasma concentrations of propofol. After an induction dose, an infusion of 100 to 200 μg/kg/min is usually needed. The infusion rate is titrated to individual requirements and the surgical stimulus. When combined with propofol, the required infusion rate and concentration of opiates, midazolam, clonidine, or ketamine should be reduced. Because opioids alter the concentration of propofol required for adequate anesthesia, the time to awakening and recovery can be influenced by these drug combinations. Also, opioids affect both the pharmacokinetics and the pharmacodynamics of propofol. Alfentanil decreases the elimination clearance of propofol from 2.1 L/min to 1.9 L/min, the distribution clearance from 2.7 L/min to 2.0 L/min, and the peripheral volume of distribution from 179 L to 141 L. The pharmacokinetic parameters of propofol are affected by cardiac output, heart rate, and plasma alfentanil concentration. Similarly, midazolam reduces propofol’s metabolic clearance from 1.94 to 1.61 L/min, Cl 2 from 2.86 to 1.52 L/min, and Cl 3 from 0.95 to 0.73 L/min. Consequently, in the presence of both midazolam and alfentanil propofol concentrations become elevated by 20% to 30%. The infusion rate required to achieve the combination with the shortest recovery is propofol, 1 to 1.5 mg/kg followed by 140 μg/kg/min for 10 minutes followed by 100 μg/kg/min, and alfentanil, 30 μg/kg followed by an infusion of 0.25 μg/kg/min, or fentanyl, 3 μg/kg followed by 0.02 μg/kg/min.
As indicated previously, increasing age is associated with a decrease in propofol infusion requirements, whereas these requirements are larger in children and infants. The blood levels of propofol alone for loss of consciousness are 2.5 to 4.5 μg/mL, and the blood concentrations (when combined with nitrous oxide) required for surgery are 2.5 to 8 μg/mL. Similar concentrations are necessary when propofol is combined with an opioid for a total IV technique. The knowledge of these levels and of the pharmacokinetics of propofol has enabled the use of pharmacokinetic model–driven infusion systems to deliver propofol as a continuous infusion for the maintenance of anesthesia. A meta-analysis of recovery data after either propofol for maintenance or the newer volatile anesthetics indicated only minor differences in times to reach recovery goals; however, the incidence of nausea and vomiting remained significantly less frequent in the patients given propofol for maintenance of anesthesia.
Propofol can be used as a maintenance of anesthesia infusion regimen for cardiac surgery. Using reduced and titrated doses of propofol for induction of anesthesia and titrated infusion rates of 50 to 200 μg/kg/min combined with an opioid for maintenance, propofol provides intraoperative hemodynamic control and ischemic episodes similar to those with either enflurane/opioid or a primary opioid technique.
Blood propofol concentrations increase in the presence of hemorrhagic shock. Shock affects the pharmacokinetics and pharmacodynamics of propofol. Shock results in slower intercompartmental clearances and shock shifts the concentration effect relationship to the left, demonstrating a 2.7-fold decrease in the effect-site concentration required to achieve 50% of the maximal effect in the BIS. These pharmacokinetic changes may be reversed with intravenous fluid resuscitation. The propofol doses needed to reach a 50% decrease from baseline BIS, and no movement after noxious stimuli, are reduced by hemorrhagic shock by 54% and 38%, respectively. Hemorrhagic shock decreases the effect-site concentration that produced 50% of the maximal BIS effect from 11.6 ± 3.8 to 9.1 ± 1.7 μg/mL and that producing a 50% probability of movement from 26.8 ± 1.0 to 20.6 ± 1.0 μg/mL.
Sedation
Propofol has been evaluated for sedation during surgical procedures and for patients receiving mechanical ventilation in the ICU. [CR] As noted earlier, tolerance can occur with propofol. Increased propofol requirement occurs with repeated anesthetic administration in a limited time period in individual patients and an increased infusion requirement when propofol is infused for prolonged periods. Infusion rates required for sedation to supplement regional anesthesia in healthy patients are half or less than the rates required for general anesthesia (i.e., 30-60 μg/kg/min). In elderly patients (>65 years old) and in sicker patients, the infusion rates that are necessary are markedly reduced up to 50% compared to 20-year-old patients. The infusion should be individually titrated to the desired effect. The pharmacokinetic profile of propofol makes it a suitable choice for long-term (days) sedation. This should always be weighed, though, against the hemodynamic side effects, tolerance, and rare occurrences of hypertriglyceridemia (and potential pancreatitis) or propofol infusion syndrome. Maintaining the smallest possible dose required for the desired level of sedation with potential “sedation holidays” should be considered as part of a long-term propofol sedation regimen. In addition, the FDA has specifically recommended against the use of propofol for the prolonged sedation of pediatric patients. The sedation guidelines of the American College of Critical Care Medicine also recommend “that patients receiving propofol for long-term sedation should be monitored for unexplained metabolic acidosis or arrhythmias. Drugs other than propofol should be considered for patients with escalating vasopressor or inotrope requirements or cardiac failure during large-dose propofol infusions.” The recommended maximal dose of propofol infusion rate is 80 μg/kg/min (<5 mg/kg/h). Generally, at propofol infusion rates more rapid than 30 μg/kg/min, patients are amnesic.
Side Effects and Contraindications
In December 2016 the FDA issued a warning raising concerns about the potential risks to fetal brain development with general anesthetics, including propofol. Animal studies show that prolonged or repetitive propofol exposure to the developing fetal brain may be related to propofol neurotoxicity. Minimizing fetal exposure to propofol and other general anesthetics is therefore important and advisable. 121a,121b
Next to its hypnotic effects, accumulating evidence suggests that propofol may affect cancer development through direct and indirect manners. Propofol exerts anti-tumor potential partly due to regulation of the expression and transfer of miRNAs. In addition, propofol impacts the degree of immunosuppression by modulating immune cells and cytokines. This results in reduced cancer cell mobility in some cancers and increased apoptosis of cancer cells in others. The clinical impact of the cancer-modulating effects of propofol needs further investigation. [CR] Induction of anesthesia with propofol is often associated with pain on injection, myoclonus, apnea, hypotension, and, rarely, thrombophlebitis of the vein into which propofol is injected. Pain on injection is reduced by using a large vein, avoiding veins in the dorsum of the hand, and adding lidocaine to the propofol solution or changing the propofol formulation. Multiple other drugs and distraction techniques have been investigated to reduce the pain on injection of propofol. Pretreatment with a small dose of propofol, opiates, nonsteroidal anti-inflammatory drugs, ketamine, esmolol/metoprolol, magnesium, a flash of light, clonidine/ephedrine combination, dexamethasone, and metoclopramide all have been tested with variable efficacy.
Propofol infusion syndrome is a rare but lethal syndrome associated with infusion of propofol at 4 mg/kg/h or more for 48 hours or longer. Yet, cases have been reported with smaller dosage schemes given for only 3 hours. It was first described in children, but subsequently has been observed in critically ill adults. The clinical features of propofol infusion syndrome are acute refractory bradycardia leading to asystole, in the presence of one or more of the following: metabolic acidosis (base deficit >10 mmol/L −1 ), rhabdomyolysis, hyperlipidemia, and enlarged or fatty liver. Other features include cardiomyopathy with acute cardiac failure, skeletal myopathy, hyperkalemia, hepatomegaly, and lipemia. The symptoms and signs are the result of muscle injury and of the release of intracellular toxic contents. The major risk factors for its development are poor oxygen delivery, sepsis, serious cerebral injury, and large propofol dosage. Predisposing factors for the propofol infusion syndrome are likely genetic disorders impairing fatty acid metabolism, such as medium-chain acyl CoA (MCAD) deficiency and low carbohydrate supply. Because lipemia has been noted, a failure of hepatic lipid regulation, possibly related to poor oxygenation or a lack of glucose, may be the cause. In some cases, an increasing lipemia was the first indication of impending propofol infusion syndrome onset, so lipemia is a sign.
Barbiturates
History
Barbiturates were discovered in the early twentieth century. The first barbiturate to cause loss of consciousness within one arm-brain circulation time was hexobarbital. After the clinical introduction of thiopental by Waters and Lundy in 1934, thiopental became preferred clinically because of its rapid onset of action and short duration, without the excitatory effects of hexobarbital. Although criticized after many casualties during the attack on Pearl Harbor as “the cause of more fatal casualties among the servicemen at Pearl Harbor than were the enemy bombs,” the barbiturates continued to be widely used in clinical practice. Many other barbiturate derivatives have been synthesized throughout the past decades, yet none have enjoyed the clinical success and popularity of thiopental.
Physicochemical Characteristics
Chemistry and Formulation
Barbiturates are hypnotically active drugs that are derivatives of barbituric acid (2,4,6-trioxohexahydropyrimidine), a hypnotically inactive pyrimidine nucleus that is formed by the condensation of malonic acid and urea ( Fig. 23.5 ). The two major classes of barbiturates are the oxybarbiturates and thiobarbiturates with either an oxygen at position 2 or with a sulfur in position 2, respectively. Through keto-enol tautomerization, the oxygen or sulfur in position 2 becomes a reactive species in the enol form. This allows for the formation of water-soluble barbiturate salts in alkaline solutions and permits the IV use of barbiturates. Although tautomerization to the enol form allows for the creation of salts, the substitution of the hydrogen attached to the carbon atom in position 5 with aryl or alkyl groups gives the barbiturates their hypnotic activity. Only thiopental and thiamylal, thiobarbiturates, and methohexital, an oxybarbiturate, have been used for induction of anesthesia ( Fig. 23.6 ). The formulation of barbiturates involves preparation as sodium salts (mixed with 6% by weight anhydrous sodium carbonate) and then reconstitution with either water, glucose 5%, or normal saline to produce a 2.5% solution of thiopental, a 2% solution of thiamylal, or a 1% solution of methohexital. The thiobarbiturates are stable for 1 week if refrigerated after reconstitution, and methohexital remains available for use for 6 weeks after reconstitution. Barbiturates cannot be reconstituted with lactated Ringer solution or mixed with other acidic solutions, as a decrease in the alkalinity of the solution can result in precipitation of the barbiturates as free acids. Examples of drugs that are not to be coadministered or mixed in solution with the barbiturates are atracurium, vecuronium, rocuronium, suxamethonium, alfentanil, sufentanil, dobutamine, dopamine, esketamine, and midazolam. Mixing of thiopental with vecuronium or pancuronium results in the formation of precipitate that may occlude the IV line during a rapid sequence induction of anesthesia.


Structure-Activity Relationships
As noted earlier, substitutions at the 5, 2, and 1 positions confer different pharmacologic activities to the barbiturate nucleus. Substitutions at position 5 with either aryl or alkyl groups produce hypnotic and sedative effects, whereas substitution at C5 with a phenyl group produces anticonvulsant activity. An increase in length of one or both side chains of an alkyl group at C5 increases hypnotic potency. Barbiturates used in clinical practice have either an oxygen or sulfur at C2. Substitution of a sulfur at position 2 produces a more rapid onset of action, as with thiopental. The addition of a methyl or ethyl group at position 1 also may produce a more rapid onset of action, as with methohexital. However, excitatory side effects, including tremor, hypertonus, and involuntary movement, may occur upon administration.
Pharmacokinetics
Metabolism
The barbiturates (with the exception of phenobarbital) are metabolized hepatically. The metabolites are almost all inactive, water-soluble, and excreted in the urine. Barbiturates are biotransformed by four processes: (1) oxidation of the aryl, alkyl, or phenyl moiety at C5; (2) N -dealkylation; (3) desulfuration of the thiobarbiturates at C2; and (4) destruction of the barbituric acid ring. Oxidation is the most important pathway, producing polar (charged) alcohols, ketones, phenols, or carboxylic acids. These metabolites are readily excreted in the urine or as glucuronic acid conjugates in the bile. Hydrolytic cleavage of the barbituratic acid ring forms a minimal contribution to the total metabolism of barbiturates, since the ring is stable in vivo. Drugs that induce oxidative microsomes or long-term administration enhance the metabolism of barbiturates. The hepatic enzyme induction by barbiturates is the reason that they are not recommended for administration to patients with acute intermittent porphyria. Barbiturates may precipitate an attack by stimulating γ-aminolevulinic acid synthetase, the enzyme responsible for the production of porphyrins.
As mentioned earlier, hepatic metabolism accounts for the elimination of all of the barbiturates with the exception of phenobarbital. Renal excretion accounts for 60% to 90% of phenobarbital excretion in an unchanged form. The alkalinization of urine with bicarbonate enhances the renal excretion of phenobarbital. Other barbiturates are excreted unchanged by the kidney only in trivial amounts.
Methohexital is metabolized in the liver by oxidation to an alcohol and N -dealkylation. Methohexital exhibits similar distribution half-lives, volumes of distribution, and protein bindings as thiopental. A marked difference exists, however, in plasma disappearance and elimination half-lives (4 hours for methohexital and 12 hours for thiopental). This difference is due to the threefold more rapid rate of hepatic clearance of methohexital (mean 7.8-12.5 mL/kg/min). The hepatic extraction ratio of methohexital (clearance to hepatic blood flow) is approximately 0.5, indicating that the liver extracts 50% of the drug presented to it. This ratio differs from the smaller hepatic extraction ratio of thiopental (0.15).
Barbiturate pharmacokinetics have been described in physiologic and compartmental models. Both of these pharmacokinetic models describe rapid redistribution as the primary mechanism that terminates the action of a single induction dose. Physiologic models of barbiturates describe a rapid mixing of the drug within the central blood volume followed by a quick distribution of the drug to highly perfused, low-volume tissues (i.e., brain) with a slower redistribution of the drug to lean tissue (muscle), which terminates the effect of the initial (induction of anesthesia) dose. In these models, adipose tissue uptake and metabolic clearance (elimination) play only a minor role in the termination of the effects of the induction dose. This is a result of the minimal perfusion ratio of adipose tissue compared with other tissues and the slow rate of removal. Compartmental model values for thiopental and methohexital, the most commonly used barbiturates for induction of anesthesia, are given in Table 23.1 . The compartmental model explains the delay of recovery when a continuous infusion of a barbiturate is used. This model describes how the termination of effect becomes increasingly dependent on the slower process of uptake into and redistribution from adipose tissue and elimination clearance through hepatic metabolism. After prolonged infusions, the pharmacokinetics of barbiturate metabolism is best approximated by nonlinear Michaelis-Menten metabolism. In usual doses (4-5 mg/kg), thiopental exhibits first-order kinetics (i.e., a constant fraction of drug is cleared from the body per unit time); however, at very high doses of thiopental (300-600 mg/kg) with receptor saturation, zero-order kinetics occur (i.e., a constant amount of drug is cleared per unit time). The volume of distribution is slightly larger in female patients, causing a longer elimination half-life. Pregnancy also increases the volume of distribution of thiopental, prolonging the elimination half-life. Even at advanced stages of liver cirrhosis, the clearance of thiopental is not altered. Because of its lipophilicity, relatively large volume of distribution, and low rate of hepatic clearance, thiopental can accumulate in tissues, especially if given in large doses over a prolonged period. The plasma drug level increases when repeated doses of drug are given. Although not used in routine clinical practice, appropriately designed infusion schemes ensure constant blood levels, maintaining the desired hypnotic effect.
Pharmacology
Mechanism of Action
The mechanisms of action of barbiturates on the CNS are largely unknown, with the exception of their action on the GABA A receptor. Perhaps the NMDA receptors are involved with the effects of barbiturates. The effects of barbiturates on the CNS have been grouped into two categories: (1) enhancement of the synaptic actions of inhibitory neurotransmitters, and (2) blockade of the synaptic actions of excitatory neurotransmitters. GABA is the principal inhibitory neurotransmitter in the mammalian CNS, and the GABA A receptor is the only site proven to be involved in barbiturate-induced anesthesia. The GABA A receptor is a chloride ion channel, composed of five subunits, with specific sites of action for GABA, barbiturates, benzodiazepines, and other molecules. The binding sites are at the interface of the specific subunits, where the combination of the adjacent subunits determine the affinity and selectivity for drugs like propofol, etomidate, or pentobarbital. Of each subunit multiple types exist, leading to various compositions of the GABA A receptor. Progressive insights in the composition of these binding sites may be useful in developing novel clinical anesthetics. [CR] Barbiturate binding to the GABA A receptor enhances and mimics the action of GABA by increasing chloride conductance through the ion channel. This causes hyperpolarization of the cell membrane and increases the threshold of excitability of the postsynaptic neuron. At low concentrations barbiturates enhance the effects of GABA, decreasing the rate of dissociation of GABA from its receptor and increasing the duration of GABA-activated chloride ion channel openings. This enhancement of the action of GABA is likely responsible for the sedative-hypnotic effects of the barbiturates. At larger concentrations, the barbiturates activate the chloride channels directly, without the binding of GABA, acting as the agonist itself. The GABA-mimetic effect at slightly higher concentrations may be responsible for what is termed barbiturate anesthesia.
The second mechanism of action of barbiturates involves the inhibition of the synaptic transmission of excitatory neurotransmitters, such as glutamate and acetylcholine. The actions of the barbiturates to block excitatory CNS transmission are specific for synaptic ion channels. Thiopental, however, may exert GABA-independent effects on the glutaminergic-NMDA system. In two studies on effects in the rat prefrontal cortex, thiopental decreased extracellular glutamate levels in the CNS and decreased NMDA-gated currents in a concentration-dependent manner.
Effects on Cerebral Metabolism (Also see Chapter 57 )
Barbiturates, like other CNS depressants, have potent effects on cerebral metabolism. A dose-related depression of cerebral metabolic oxygen consumption rate (CMRO 2 ) progressively slows the EEG, reduces the rate of adenosine triphosphate consumption, and enhances protection from partial cerebral ischemia. The relationship between depressed metabolism and drug requirement was shown when thiopental was not eliminated (i.e., sustained with an extracorporeal circulation pump). When the EEG became isoelectric, a point at which cerebral metabolic activity is roughly 50% of baseline, no further decreases in CMRO 2 occurred. These findings support the hypothesis that metabolism and function of the brain are coupled. Barbiturates reduce the metabolic activity concerned with neuronal signaling and impulse traffic, not the metabolic activity corresponding to basal metabolic function. The only way to suppress baseline metabolic activity concerned with cellular activity is via hypothermia. The effect of barbiturates on cerebral metabolism is maximized at a 50% depression of cerebral function, leaving all metabolic energy for the maintenance of cellular integrity. With the reduction in CMRO 2 , there is a parallel reduction in cerebral perfusion, which is seen in decreased cerebral blood flow (CBF) and ICP. With reduced CMRO 2 , cerebral vascular resistance increases, and CBF decreases. The ratio of CBF to CMRO 2 remains unchanged. Even though the mean arterial pressure (MAP) decreases, barbiturates do not compromise the overall CPP because the CPP equals MAP minus ICP. In this relationship, ICP decreases more than MAP after barbiturate administration, preserving CPP.
Pharmacodynamics
Sufficient doses of barbiturates cause loss of consciousness, amnesia, and respiratory and cardiovascular depression, known as general anesthesia. The response to pain and other noxious stimulation during general anesthesia seems to be depressed. Pain studies reveal that barbiturates may decrease the pain threshold. This antianalgesic effect occurs only with low blood levels of barbiturates, which might be achieved with small doses of thiopental for induction of anesthesia or after emergence from thiopental. The amnesic effect of barbiturates is less pronounced than that produced by benzodiazepines.
Effects on the Central Nervous System
Drugs with high lipid solubility and a low degree of ionization cross the blood-brain barrier rapidly, producing a fast onset of action. Most barbiturates exist in a nonionized form. Thiopental and methohexital are more lipid soluble than pentobarbital, which is clinically reflected by the more rapid onset of action of thiopental and methohexital compared to pentobarbital. Only the nonionized form of a drug can directly traverse the cellular membranes. Thiopental has a p K a of 7.6. Approximately 50% of thiopental is nonionized at physiologic pH, which accounts partly for the rapid accumulation of thiopental in the cerebrospinal fluid (CSF) after IV administration. Methohexital is 75% nonionized at pH 7.4, which may explain the slightly more rapid effect of methohexital compared to thiopental. As pH decreases, with poor perfusion, barbiturates have a larger fraction of nonionized drug available to cross the blood-brain barrier.
The onset of action in the CNS is affected by protein binding, because only unbound drug (free drug) can cross the blood-brain barrier. Barbiturates are highly bound to albumin and other plasma proteins, where the thiobarbiturates are more highly protein bound than are the oxybarbiturates. The degree of protein binding of a drug is influenced by the physiologic pH and disease states, which alter the absolute amount of protein. Most barbiturates tend to experience peak protein binding at or around pH 7.5. The final factor governing the rapidity of drug penetration of the blood-brain barrier is the plasma drug concentration, causing a concentration gradient. The two primary determinants of the plasma concentration are the dose administered and the rate (speed) of administration. As the dose of thiopental over the same time is increased, an increased percentage of patients will be anesthetized. Concerning absolute dose, 2 mg/kg produced anesthesia in 20% of patients, whereas a dose of 2.4 mg/kg produced anesthesia in 80% of patients. Similarly, the speed of injection influences the effect of thiopental. A smaller amount of drug is required to produce anesthesia when the dose rate of the administration is over 5 seconds as opposed to over 15 seconds.
Because of the equilibrium between brain concentration and plasma concentration, factors that determine the rate of onset of barbiturate effects also affect their termination. Lipid solubility, degree of ionization, and CSF drug concentration affect the movement of drug from the CSF to plasma. As plasma levels decrease, drug levels in the brain and CSF decrease. The most important factors in the termination of drug effect are those that govern plasma disappearance of the drug. These are generally divided into a rapid redistribution phase and a slow metabolic and second redistribution phase. In a classic pharmacologic study, Brodie and coworkers showed that awakening from thiopental occurred because the plasma concentration rapidly declined. They further showed that the cause of the rapid plasma decay of thiopental was not metabolism of the drug but was rather due to a redistribution of the drug to other tissues throughout the body. The relationship of the plasma drug level and drug redistribution to the onset and termination of effect is illustrated in Fig. 23.7 . Clinically, patients awake from a single dose of thiopental 5 to 10 minutes after administration, as the drug is redistributed from highly perfused CNS tissues to well-perfused lean tissues. The termination of effect after multiple drug administrations or constant infusion depends on the elimination of the drug from the blood, which becomes increasingly more dependent on first-order metabolism than redistribution and is a function of its context-sensitive decrement time (see Fig. 23.3 ). Awakening may be delayed in older patients because of increased CNS sensitivity, alterations in metabolism, or decreased central volume of distribution relative to younger adults. The initial volume of distribution is less in elderly patients than in young patients, which explains a smaller dose requirement for the onset effect. Pediatric patients (<13 years old) seem to have a more rapid rate of total clearance and a shorter rate of plasma thiopental clearance than do adults, which theoretically might result in earlier awakening, especially after multiple doses of the drug. There is little difference in distribution of thiopental and methohexital, which may explain the similar wakeup times. There is, however, a difference in the rate of total body clearance, being more rapid for methohexital. This disparity explains the difference found in the psychomotor skills of patients and the earlier full recovery after methohexital. Despite some residual effects, methohexital is cleared more rapidly than thiopental, which explains why methohexital is preferred for use by some clinicians when rapid awakening is desirable, such as in outpatient anesthesia. Prolongation of early and late recovery by the barbiturates is the primary reason behind the fact that they have been largely replaced by propofol.

Effects on the Respiratory System
Barbiturates produce dose-related central respiratory depression. The evidence for central depression is a correlation between EEG suppression and diminished minute ventilation. Peak respiratory depression (as measured by the slope of carbon dioxide concentration in the blood) and maximum depression of minute ventilation after delivery of thiopental (3.5 mg/kg) occurs 1 to 1.5 minutes after administration. These variables return to predrug levels rapidly, and within 15 minutes the drug effects are barely detectable. Patients with chronic lung disease are slightly more susceptible to the respiratory depression of thiopental. The ventilatory pattern with thiopental induction has been described as “double apnea,” that is an initial apnea of a few seconds occurring upon drug administration, succeeded by a few breaths of reasonably adequate tidal volume, which is followed by a more prolonged period of apnea, typically of approximately 25 seconds. This apnea occurs in at least 20% of cases. During the induction of anesthesia with thiopental, ventilation must be assisted or controlled to provide adequate respiratory exchange. Like thiopental, methohexital is a central respiratory system depressant. Induction doses (1.5 mg/kg) significantly decrease the slope of the ventilatory response to carbon dioxide, with a maximal reduction at 30 seconds after drug administration. The peak decrease in tidal volume occurs 60 seconds after methohexital administration. All variables return to baseline within 15 minutes. In contrast to the effects on ventilation, patients awaken within about 5 minutes after the administration of methohexital (1.5 mg/kg).
Effects on the Cardiovascular System
Cardiovascular depression from barbiturates is a result of central and peripheral (direct vascular and cardiac) effects. The primary cardiovascular effect of a barbiturate during induction of anesthesia is peripheral vasodilation causing a pooling of blood in the venous system. Mechanisms for the decrease in cardiac output include (1) direct negative inotropic action, due to a decrease of calcium influx into the cells, (2) decreased ventricular filling, due to increased capacitance, and (3) transiently decreased sympathetic outflow from the CNS. The increase in heart rate (10% to 36%) that accompanies thiopental administration probably results from the baroreceptor-mediated sympathetic reflex stimulation of the heart in response to the decrease in output and pressure. The cardiac index, as well as the MAP, is unchanged or reduced. Hemodynamic changes are dependent on the infusion rate of thiopental. In the dose ranges hitherto studied, no relationship between plasma thiopental level and hemodynamic effect has been found. There is little difference in the responses after thiopental and methohexital administration in patients with heart disease. The increase in heart rate (11%-36%) encountered in patients with coronary artery disease anesthetized with thiopental (1-4 mg/kg) is potentially deleterious because of the obligatory increase in myocardial oxygen consumption that accompanies the increased heart rate. In a study in dogs, thiopental prolonged the QT interval, flattened T waves, and increased QT dispersion during and after induction. Thiopental may not be the most appropriate choice for patients with a susceptibility to ventricular dysrhythmias or a prolonged QT interval, such as acidotic patients or conditions that prolong the QT interval, such as long-term dialysis or advanced cirrhosis. Patients who have normal coronary arteries have no difficulty in maintaining adequate coronary blood flow to meet the increased myocardial oxygen consumption. Thiopental should be avoided in hypovolemic patients because there is a significant reduction in cardiac output (69%) and a significant decrease in arterial blood pressure. Patients without adequate compensatory mechanisms may have serious hemodynamic depression with a thiopental induction of anesthesia.
Other Effects
The side effects of injecting barbiturates include a garlic or onion taste (40% of patients), allergic reactions, local tissue irritation, and rarely, tissue necrosis. A transient urticarial rash may develop on the head, neck, and trunk. More severe reactions such as facial edema, hives, bronchospasm, and anaphylaxis can occur. Treatment of anaphylaxis is symptomatic. Thiopental and thiamylal produce fewer excitatory symptoms with induction than methohexital, which produces cough, hiccups, tremors, and twitching approximately five times more often. Tissue irritation and local complications may occur more frequently with the use of thiopental and thiamylal than with methohexital.
The consequences of accidental arterial injection may be severe. The degree of injury is related to the concentration of the drug. Treatment consists of (1) dilution of the drug by the administration of saline into the artery, (2) heparinization to prevent thrombosis, and (3) brachial plexus block. Overall, the proper administration of thiopental intravenously is remarkably free of local toxicity.
Phenobarbital is used experimentally as an inducer of the cytochrome P450 (CYP) system in rodents, in particular of the CYP2B enzymes. In human hepatocyte culture, phenobarbital acts as an inducer of the CYP2B6, CYP2C9, CYP2C19, and CYP3A4 enzymes. This phenomenon may cause changes in the metabolism of drugs that are administered concomitantly. On the other hand, the metabolism of thiopental can be influenced by concomitant drugs like selective serotonin reuptake inhibitor s (SSRIs). These drugs are used frequently by patients who are treated by electroconvulsive therapy, in whom an induction with thiopental or thiamylal is performed.
Uses
Induction and Maintanance of Anesthesia
Barbiturates are used clinically for induction and maintenance of anesthesia and premedication. Methohexital is the drug of choice for providing anesthesia during electroconvulsive therapy. Other barbiturates used in this field are thiopental and thiamylal. Less frequently, barbiturates are used to provide cerebral protection in patients at risk of developing incomplete ischemia. The three barbiturates that are used most commonly for IV anesthesia induction and maintenance are thiopental, thiamylal, and methohexital. Thiopental is an excellent drug to use for induction of anesthesia. The prompt onset (15-30 seconds) of action and smooth induction are advantages for this drug. The rapid emergence, particularly after single use for induction, also was a reason for the widespread use of thiopental in this setting. Thiopental can be used to maintain general anesthesia because repeated doses reliably sustain unconsciousness and contribute to amnesia but should not be the drug of first choice as the hypnotic component in balanced anesthesia. A review of the role of anesthetics on the risk of awareness during surgery show that benzodiazepines reduce awareness compared to thiopental, ketamine, and placebo. Wakefulness is reduced by ketamine and etomidate, compared with thiopental. The evidence is not strong due to the risk of bias, the very small event rate, and the heterogeneity in definition of awareness.
Methohexital is the only IV barbiturate used for induction of anesthesia that can compete with thiopental. With a dose of 1 to 2 mg/kg, induction and emergence from anesthesia is rapid. Methohexital also may be used as a hypnotic component to maintain anesthesia. Similar to thiopental, it is not an analgesic. Additional opioids or volatile anesthetics are required to provide a balanced technique satisfactory for general anesthesia during surgery. Because methohexital is cleared more rapidly than thiopental, it may be preferred to thiopental for the maintenance of anesthesia, as accumulation and saturation of peripheral sites takes longer. For brief infusion (<60 minutes), recovery from a methohexital infusion titrated to maintain hypnosis (50-150 μg/kg/min) is similar to that provided by propofol. There are probably upper limits of safe infusion doses yet to be defined, but seizures have occurred in neurosurgical patients after large doses of methohexital (24 mg/kg). Methohexital may be given rectally and is absorbed rapidly, and can be used as a premedication drug in pediatric patients. The dose recommended for this use is 25 mg/kg rectal instillation (10% solution through a 14F catheter, 7 cm into rectum). With this method of administration, sleep onset is rapid: mean peak plasma levels occur within 14 minutes.
Dosing
Dosing for the two most commonly used barbiturates is listed in Table 23.4 . The usual doses of thiopental (3-4 mg/kg) and thiamylal (3-4 mg/kg) are about twice the dose of methohexital (1-2 mg/kg). In dose-response studies, the ED 50 for thiopental ranged from 2.2 to 2.7 mg/kg, and the ED 50 for methohexital was 1.1 mg/kg. There is less interpatient variability in the dose response to barbiturates than to benzodiazepines when used for anesthesia induction, but there still is significant variability in the dose of thiopental required to induce anesthesia. Interpatient dose variability is related to the presence of hemorrhagic shock, cardiac output, lean body mass, obesity, gender, and age. Hemorrhagic shock, lean body mass, age, and obesity explain variability of patient response owing to a decrease in the central volume of distribution. Finally, patients who have severe anemia, burns, malnutrition, widespread malignant disease, uremia, ulcerative colitis, or intestinal obstruction also require smaller induction doses of barbiturates.
Drug | Induction Dose (mg/kg) ∗ , † | Onset (s) | Intravenous Maintenance Infusion |
---|---|---|---|
Thiopental | 3-4 | 10-30 | 50-100 mg every 10-12 min |
Methohexital | 1-1.5 | 10-30 | 20-40 mg every 4-7 min |
∗ Adult and pediatric intravenous doses are roughly the same in milligrams per kilogram.
† Methohexital can be given rectally in pediatric patients as a 20 to 25 mg/kg/dose.
Contraindications
The following conditions should be considered a contraindication for the use of IV barbiturates: (1) when there is respiratory obstruction or an inadequate airway, thiopental may worsen respiratory depression; (2) severe cardiovascular instability or shock contraindicate its use; (3) status asthmaticus is a condition in which airway control and ventilation may be worsened further by thiopental; (4) porphyria may be precipitated or acute attacks may be accentuated by the administration of thiopental; and (5) without proper induction equipment (IV instrumentation) and airway equipment (means of artificial ventilation), thiopental should not be administered.
Benzodiazepines
Introduction
The benzodiazepines have become a category of drugs widely used in anesthesia as anxiolytics, sedatives, and hypnotics. They exert their action through GABA A receptors, which are the key targets that mediate most of the clinically important effects of IV anesthetics. In the clinical practice of daily anesthesia, midazolam is often used immediately before induction of anesthesia. The other agonists, diazepam, lorazepam, temazepam, and the antagonist flumazenil are sometimes used. Remimazolam, an ultrashort-acting GABA A receptor agonist, may be a useful new benzodiazepine in future anesthetic practice. Benzodiazepines are widely prescribed, and addiction to these drugs is a worldwide concern. Research is ongoing to elucidate the neural mechanism of the reward-related effects of benzodiazepines. Reynolds and associates conclude in their findings that α 2 – and α 3 -subunit–containing GABA A receptors are implicated as key mediators of the reward-related effects of benzodiazepines. This finding has important implications for the development of new drugs with less addictive properties.
Surgical treatment of tumors is often the first line for several types of cancer. Several factors influence the metastatic spread of residual cancer cells. Midazolam is widely used during general anesthesia. Research in vitro and in vivo conclude that midazolam in contrast to dexmedetomidine has antitumorigenic properties in certain types of cancer in supraclinical dosage.
Benzodiazepines were discovered in 1954 by Sternbach and in 1959 chlordiazepoxide (Librium) was the first benzodiazepine patented. Diazepam was synthesized in 1963 in a search for a better compound and was used intravenously to induce anesthesia in 1965. Oxazepam (Seresta), a metabolite of diazepam, was synthesized in 1961 by Bell. Lorazepam (Ativan), a 2′chloro-substitution product of oxazepam, was synthesized in 1971 in an attempt to produce a more potent benzodiazepine. The next major achievement was Fryer and Walser’s synthesis in 1976 of midazolam (Versed, Dormicum), the first clinically used water-soluble benzodiazepine, produced primarily for use in anesthesia. An ultrashort-acting benzodiazepine, remimazolam, is in its phase III trial and is promising.
Physicochemical Characteristics
Four benzodiazepine receptor agonists are commonly used in anesthesia: midazolam, diazepam, lorazepam, and temazepam ( Fig. 23.8 ). The physicochemical characteristics of the benzodiazepines used in anesthesia are listed in Table 23.5 . These six molecules are relatively small and are lipid soluble at physiologic pH.

Molecular Weight | pK a | Water Solubility | Lipid Solubility | |
---|---|---|---|---|
Da | g/L | Log P | ||
Diazepam | 284.7 | 3.4 | 0.051 | 2.801 |
Lorazepam | 321.2 | 1.3 | 0.12 | 2.382 |
Temazepam | 300.7 | 1.6, 11.7 | 0.28 | 2.188 |
Midazolam | 325.8 (hydrochloride 362.2) | 6.0 | 0.004 (2.0, pH 1) | 3.798 |
Remimazolam | 439.3 (besylate 597.5) | 5.3 | 0.008 (7.5, pH 1) | 3.724 |
Flumazenil | 303.3 | 0.86 | 0.042 | 2.151 |
Of the clinically used benzodiazepines, midazolam is the most lipid soluble in vivo, but because of its pH-dependent solubility, it is water soluble when formulated in a buffered acidic medium (pH 3.5). The imidazole ring of midazolam accounts for its stability in solution and its lipophilicity due to rapid closing of the imidazole ring at physiological pH. The high lipophilicity of the compounds accounts for the rapid CNS effect and relatively large volumes of distribution.
Pharmacokinetics
The four benzodiazepines used in anesthesia are classified as short-acting (midazolam), intermediate-acting (lorazepam, temazepam), and long-acting (diazepam), according to their metabolism and plasma clearance ( Table 23.6 ). The plasma disappearance curves of all benzodiazepines can be best described by a two- or three-compartment model.
Eliminationhalf-life (h) | Clearance (mL/kg/min) | Vd (L/kg) | Plasma Protein Binding (%) | Reference | |
---|---|---|---|---|---|
Midazolam | 1.7-3.5 | 5.8-9.0 | 1.1-1.7 | 94-98 | Dundee et al. (1984) |
Diazepam | 20-50 | 0.2-0.5 | 0.7-1.7 | 98-99 | Greenblatt et al. (1980) |
Lorazepam | 11-22 | 0.8-1.5 | 0.8-1.3 | 88-92 | Greenblatt et al. (1979) |
Temazepam | 6-8 | 1.0-1.2 | 1.3-1.5 | 96-98 | Fraschini and Stankov (1993) |
Remimazolam ∗ | 0.4 | 4521 mL/min | 36.4 L | N.A. | Upton et al. (2010) |
Flumazenil | 0.7-1.3 | 13-17 | 0.9-1.9 | 40-50 | Klotz and Kanto (1998) |
Protein binding and volumes of distribution are not much different among these four benzodiazepines, but the clearance is significantly different. Factors that may influence the pharmacokinetics of benzodiazepines are age, gender, race, enzyme induction, and hepatic and renal disease. In addition, the pharmacokinetics of the benzodiazepines are affected by obesity. The volume of distribution is increased as drug diffuses from the plasma into the adipose tissue. Although clearance is not altered, elimination half-lives are prolonged, owing to the increased volume of distribution and delayed return of the drug to the plasma in obese patients. Generally, sensitivity to benzodiazepines in some patient groups, such as elderly patients, is greater despite relatively modest pharmacokinetic changes; factors other than pharmacokinetics must be considered when these drugs are used.
Midazolam : After oral ingestion midazolam is absorbed completely and the peak plasma concentration is achieved in 30 to 80 minutes. The bioavailability is less than 50% due to a significant first-pass metabolism in the intestinal wall and the liver. After IV administration, midazolam is rapidly distributed, with a distribution half-life of 6 to 15 minutes. The plasma protein binding is high: 94% to 98%.
The hepatic extraction ratio is low, ranging from 0.30 to 0.44, but is higher than the unbound free fraction of midazolam in plasma. Thus, the protein binding is not a restrictive factor in drug extraction by the liver. With this intermediate hepatic extraction ratio, the metabolic clearance of midazolam may be susceptible both to changes in enzyme activity and to changes in hepatic blood flow.
The elimination half-life ranges from 1.7 to 3.5 hours. The plasma clearance ranges from 5.8 to 9.0 mL/kg/min and is higher compared to that of the other benzodiazepines. This is due to the fused imidazole ring, which is rapidly oxidized in vivo—much more rapidly than the methylene group of the diazepine ring of other benzodiazepines.
The pharmacokinetics of midazolam are affected by obesity, age, hepatic cirrhosis, and severity of critical illness. Because of its high lipophilicity (at physiological pH), midazolam distributes preferably to adipose tissue resulting in a prolonged elimination half-life in obese patients. Liver cirrhosis reduces plasma clearance of midazolam due to decreased metabolism.
Midazolam is metabolized by CYP3A4 and CYP3A5 to 1-hydroxymethylmidazolam (= α-hydroxymidazolam) and 4-hydroxymidazolam. These metabolites possess similar sedative activities compared to the parent compound and, when given over a longer time, these metabolites may accumulate. These metabolites are rapidly conjugated and excreted in the urine and have, like midazolam, a marked increase in peripheral volume of distribution in obese/overweight adolescents. In comparison, 1-hydroxymethylmidazolam is less potent than its parent compound, the affinity to the receptor is about 60%, and weaker than that of midazolam. The metabolites are cleared more rapidly than midazolam itself, making them of little concern in patients with normal hepatic and renal function. In patients with renal impairment, though, they can cause profound sedation.
Diazepam : After oral ingestion the bioavailability is about 94%. Time to peak plasma concentrations after oral ingestion is approximately 60 minutes. Diazepam is extensively bound to plasma proteins; the volume of distribution ranges from 0.7 to 4.7 L/kg. The plasma clearance of diazepam ranges from 0.2 to 0.5 mL/kg/min.
The pharmacokinetics of diazepam are affected by obesity and liver dysfunction and particularly by age. Increasing age reduces the clearance of diazepam significantly.
Metabolism occurs in the liver and is mediated mainly by CYP2C19 and CYP3A4. This accounts for 80% of the biotransformation of diazepam. The metabolite N-desmethyldiazepam has pharmacodynamic characteristics similar to those of diazepam, but has a much slower elimination half-life extending to 200 hours. N-desmethyldiazepam is further metabolized to oxazepam, which is also pharmacologically active.
Temazepam, another metabolite of diazepam, is mainly conjugated to temazepam glucuronide and a smaller part is demethylated to oxazepam and thereafter conjugated to oxazepam-glucuronide.
Lorazepam : The oral bioavailability is high, nearly 90%. Peak plasma concentrations are reached approximately 2 hours after oral ingestion, the mean elimination half-life is 15 hours, with a range of 8 to 25 hours. Lorazepam has a large volume of distribution from 0.8 to 1.3 L/kg, and it is highly bound to plasma proteins (>90%).
The clearance of lorazepam is 0.8 to 1.8 mL/kg/min. Lorazepam is conjugated in the liver to an inactive glucuronide and up to 70% is excreted in urine. The pharmacokinetics of lorazepam is little altered by age, and not altered by gender or renal disease, but clearance is decreased by hepatic dysfunction.
Remimazolam (CNS 7056)
Remimazolam, a new short-acting GABA A receptor agonist with high affinity to the GABA receptor, is rapidly degraded in plasma by nonspecific esterases to its carboxylic acid metabolite CNS 7054. The incorporation of a carboxylic ester moiety into the benzodiazepine core of remimazolam renders it susceptible to nonspecific tissue esterases. Preclinical studies in sheep showed a more rapid onset of action, greater depth of sedation, and more rapid recovery than midazolam. In sheep, remimazolam showed no dose-dependent depth of sedation like propofol. In humans, remimazolam is eliminated by first-order pharmacokinetics, with no clear relationship registered between body weight and elimination clearance. Accumulation after prolonged infusion is unlikely. The clearance of remimazolam was rapid (overall mean clearance 70.3 ± 13.9 L/hr) and the volume of distribution moderately large (steady-state volume of distribution is 34.8 ± 9.4 L). No clear relation exists between body weight and systemic clearance. Level and duration of sedation are dose-dependent in humans. Remimazolam trials conclude a safe administration for procedural sedation; it allows a faster recovery of neuropsychiatric function compared to midazolam. Possible benefit compared to propofol is the safe administration by endoscopists instead of healthcare providers trained in anesthesia.
Pharmacodynamics
Benzodiazepines act selectively at the GABA A receptor, which mediates fast inhibitory synaptic transmission in the CNS. Benzodiazepines enhance the response to GABA by facilitating the opening of the GABA-activated chloride channels resulting in hyperpolarization. A series of compounds are candidates as endogenous ligand of the GABA A receptor, such as diazepam binding inhibitor and other substances. This is an area of ongoing research.
Translocator protein (TSPO, 18 kDa), first described as a peripheral binding site for benzodiazepines, which are not associated with GABA receptors, are expressed throughout the body and brain. While their precise function and pharmacologic significance remain only partly known, the TSPO role has many proposed functions depending on the tissue, like cholesterol transport, regulatory role in the heart, and immunomodulation related to inflammatory activation.
Effects on the Central Nervous System
All benzodiazepines have hypnotic, sedative, anxiolytic, amnesic, anticonvulsant, and centrally produced muscle-relaxing properties. They may differ to some extent in their potency and efficacy with regard to some of these pharmacodynamic actions (e.g., anticonvulsive action). The neurotransmitter GABA is an inhibitory neurotransmitter and controls the state of a chloride ion channel. Activation of this chloride ion channel results in neuronal hyperpolarization (increased membrane potential in the direction away from the threshold potential) and accounts for the classification of the GABA system as “inhibitory.” Benzodiazepines bind to their receptors with high affinity; the binding is stereospecific and saturable; the order of receptor affinity (potency) of three agonists is lorazepam > midazolam > diazepam. Midazolam is approximately 3 to 6 times, and lorazepam 5 to 10 times, as potent as diazepam. As indicated previously, the mechanism of action of benzodiazepines is reasonably well understood. The interaction of benzodiazepine ligands with the GABA A receptor is one of the few examples in which the complex systems of biochemistry, molecular pharmacology, genetic mutations, and clinical behavioral patterns can to some extent be explained. GABA A subtypes mediate the different effects (amnesic, anticonvulsant, anxiolytic, and sleep). The GABA A receptor is a pentameric assembly built from 18 or more subunits ( Fig. 23.9 ). Many different combinations of this pentameric assembly occur in different parts of the brain; linking this diversity to physiological function and pharmacologic specificity may be possible. The α-subunit of the pentameric complex occurs in six isoforms (α 1 -α 6 ). Sedation, anterograde amnesia, and anticonvulsant properties are mediated via α 1 -subunits of the GABA A receptors, and anxiolysis and muscle relaxation are mediated via the α 2 -subunits. The “benzodiazepine receptors” are found in highest densities in the olfactory bulb, cerebral cortex, cerebellum, hippocampus, substantia nigra, and inferior colliculus, where lower densities in the striatum, lower brainstem, and spinal cord are found. Spinal cord benzodiazepine receptors can play an important role in analgesia; however, further elucidation of the mechanism of action of this drug class is required. Intrathecal midazolam reduces excitatory GABA-mediated neurotransmission in interneurons, leading to a decrease in the excitability of spinal dorsal horn neurons. A meta-analysis shows that intrathecal midazolam improves perioperative analgesia and reduces nausea and vomiting.

The benzodiazepines reduce the CMRO 2 in a dose-related manner. Midazolam and diazepam maintain a relatively normal ratio of CBF to CMRO 2 . Midazolam, diazepam, and lorazepam all increase the seizure initiation threshold to local anesthetics and decrease the mortality rate in mice exposed to lethal doses of local anesthetics. Midazolam has neuroprotective effects by preventing lipid peroxidation and mitochondrial damage. There are indications in rats that the peripheral benzodiazepine receptor is involved in these actions.
Effects on the Respiratory System
Benzodiazepines, similar to most IV anesthetics, produce dose-related central respiratory system depression. The benzodiazepines affect respiration in two different ways. First, they have an effect on the muscular tone leading to an increased risk of upper airway obstruction. Second, they flatten the response of the respiratory curve to carbon dioxide. In addition, sedative doses of midazolam depress the hypoxic ventilatory response in humans.
Benzodiazepines and opioids produce additive or supra-additive (synergistic) respiratory depression, even though they act at different receptors. Old age, debilitating disease, and other respiratory depressant drugs increase the incidence and degree of respiratory depression and apnea by benzodiazepines.
Effects on the Cardiovascular System
The hypothalamic paraventricular nucleus (PVN) is an important site for autonomic and endocrine homeostasis of the cardiovascular system. The PVN integrates afferent stimuli to regulate blood volume; the rostral ventrolateral medulla is the dominant brain region for tonic regulation of arterial blood pressure. Under normal circumstances, the sympathetic nervous system is tonically inhibited. This inhibition is dependent on GABAergic signaling and nitric oxide.
Benzodiazepines, when used alone, have modest hemodynamic effects. The predominant hemodynamic change is a modest decrease in arterial blood pressure, resulting from a decrease in systemic vascular resistance. The mechanism by which benzodiazepines maintain relatively stable hemodynamics involves the preservation of homeostatic reflex mechanisms. The hemodynamic effects of midazolam and diazepam are dose related, however, there is a plateau plasma drug effect above which little change in arterial blood pressure occurs. The plateau plasma level for midazolam is 100 ng/mL, and that for diazepam is about 900 ng/mL. Heart rate, ventricular filling pressures, and cardiac output are maintained after induction of anesthesia with benzodiazepines. More recent studies using heart rate variability variables as measurements to evaluate the effect of benzodiazepines on autonomic neurocardiac regulation conclude a biphasic effect. First, the vagal tone reduces and second, the cardiac pacemaker may decrease using intravenous premedication doses. In patients with increased left ventricular filling pressures, diazepam and midazolam produce a “nitroglycerin-like” effect by decreasing the filling pressure and increasing cardiac output. Notably, the stress of endotracheal intubation and surgery are not blocked by midazolam.
Drug Interactions
Pharmacokinetic Drug Interactions
The pharmacokinetics of benzodiazepines may be altered by drug interactions. As cytochrome P450 is often involved in the metabolism of the benzodiazepines, drugs inducing or inhibiting CYP function often cause alterations in the pharmacokinetics of the benzodiazepines.
CYP-mediated drug interactions have extensively been examined utilizing midazolam, which is almost completely metabolized by CYP, particularly CYP3A4.
The inhibition of CYP3A by concomitantly administered drugs like—among many others—the azole antifungal agents, results in significant inhibition of the metabolism of midazolam. Orally administered midazolam is especially affected by these inhibitors due to reduction of the first-pass metabolism elimination.
Diazepam is primarily metabolized by CYP2C19 and CYP3A4. Different CYP2C19 alleles have varying activity, which results in ultra-rapid, extensive, intermediate, and poor metabolizer genotypes. Pharmacokinetics and pharmacodynamics vary among these different metabolizers. Strong inhibitors of CYP3A4 have a minor effect on the pharmacokinetics of diazepam. Inhibitors of CYP2C19 like omeprazole, fluvoxamine, and ciprofloxacin consequently increase the plasma half-life of diazepam substantially. The clearance of lorazepam is affected by probenecid and valproic acid, both of which decrease the formation clearance of lorazepam-glucuronide. Remimazolam is not metabolized by CYP-dependent mechanisms, which decreases the chances of significant drug interactions occurring.
Pharmacodynamic Drug Interaction
All benzodiazepines act on the CNS and interact with other drugs targeting the CNS, in particular those causing CNS depression.
In anesthetic practice, opioids are often combined with benzodiazepines, which interact in a synergistic manner. The interaction between midazolam and ketamine is additive, while the interaction between the hypnotic effects of thiopental and midazolam and of propofol and midazolam are synergistic.
Uses
Premedication
Benzodiazepines are the most commonly applied drugs for premedication. The goals of this application are anxiolysis, sedation, amnesia, vagolysis, and sympathicolysis, and reduction of PONV. The amnestic effects are anterograde; retrograde memory is not affected.
Diazepam, lorazepam, and midazolam are given orally or intravenously for preoperative sedation. Midazolam is the most frequently used benzodiazepine for premedication in both adults and children. The usual oral dose for adults ranges from 7.5 to 15 mg for midazolam, from 5 to 10 mg for diazepam, and from 10 to 20 mg for temazepam. Many factors like age, ASA physical status, level of anxiety, and type and length of surgery determine the dose. Lorazepam is mostly used when a prolonged and intense anxiolysis is pursued, like in cardiac surgery. Typically, 2 to 4 mg lorazepam is administered orally 2 hours before anesthesia and surgery.
For pediatric patients midazolam is available in several preparations (including a formulation for intranasal administration in some countries) and well tolerated. The dose is effective from 0.025 mg/kg and produces sedation and anxiolysis in 10 to 20 minutes.
Midazolam has minimal effects on respiration and oxygen saturation in adults, even in doses up to 1.0 mg/kg with a maximum of 20 mg.
Sedation
Relief of anxiety and lack of recall of unpleasant events during minor surgical and diagnostic procedures are the primary objectives of good sedation. Appropriately used sedation improves patient satisfaction. Patients are seemingly conscious and coherent during sedation with benzodiazepines, yet they are amnesic for the procedure and events. For this use drugs should be given by titration; end points of titration are adequate sedation or dysarthria ( Table 23.7 ). The onset of action is rapid with midazolam, usually with a peak effect reached within 2 to 3 minutes of administration; time to peak effect is slightly longer with diazepam and even longer still with lorazepam. The duration of action of these drugs are dose-dependent. Although the onset is more rapid with midazolam than with diazepam after bolus administration, the recovery is similar, probably because both drugs have similar early plasma decay (redistribution) patterns. (also see Fig. 23.10 ). With lorazepam, sedation and particularly amnesia are slower in onset and are longer lasting than with the other two benzodiazepines. Lorazepam is particularly unpredictable with regard to duration of amnesia, and this is undesirable in patients who wish or need to have recall in the immediate postoperative period. The degree of sedation, the reliable amnesia, and the preservation of respiratory and hemodynamic function are better with benzodiazepines than with other sedative-hypnotic drugs used for conscious sedation. When midazolam is compared with propofol for sedation during procedures, the two are generally similar except that emergence or wake-up is more rapid with propofol. Sedation with propofol is safe in the hands of well-trained non-anesthetic professionals.
Midazolam | Diazepam | Lorazepam | |
---|---|---|---|
Induction | 0.05-0.15 mg/kg | 0.3-0.5 mg/kg | 0.1 mg/kg |
Maintenance | 0.05 mg/kg prn | 0.1 mg/kg prn | 0.02 mg/kg prn |
1 μg/kg/min | |||
Sedation ∗ | 0.5-1 mg repeated | 2 mg repeated | 0.25 mg repeated |
0.07 mg/kg IM |
∗ Incremental doses given until desired degree of sedation is obtained.

There are studies showing that remimazolam has a favorable profile as a sedative for upper gastrointestinal endoscopy in patients as the time to recovery was shorter and more consistent than that observed after the use of midazolam. The use of midazolam for sedation during regional and epidural anesthesia requires vigilance with regard to depth of sedation and respiratory function.
Two studies report the use of midazolam during cesarean section either for sedation in preeclamptic parturients or prevention of nausea and vomiting; they showed that a single IV dose of midazolam was a safe practice, with no detriment in Apgar scores, neurobehavioral scores, continuous oxygen saturation, or the ability of the mother to recall the birth events. Nitsun and associates found that 0.005% of the maternal dose of midazolam is transferred into the breast milk during a 24-hour milk collection. Although verification of these findings is needed, they highlight an important clinical use of midazolam that may be safe for mother and infant.
Sedation for longer periods, such as in the ICU, can also be accomplished with benzodiazepines. Prolonged infusion may result in accumulation of drug and, in the case of midazolam, significant concentration of the active metabolite. Reviews have pointed out both concerns and advantages of benzodiazepine sedation. The main advantages are the amnesia and hemodynamic stability, where the disadvantage is the potential lingering sedative effect after termination of the infusion when compared with propofol and a higher prevalence of delirium compared to dexmedetomidine. In 2013, the Society of Critical Care Medicine’s (SCCM) American College of Critical Care Medicine (ACCM) published a revised version of its pain, agitation, and delirium (PAD) clinical practice guidelines for adult ICU patients. These guidelines suggest that sedation strategies using nonbenzodiazepine sedatives may be preferred over sedation with benzodiazepines, either midazolam or lorazepam, to improve clinical outcome in mechanically ventilated ICU patients. To prevent overdosing and prolonged mechanical ventilation, evidence-based sedation algorithms have evolved. Daily interruption of sedation has not proven to decrease the time to extubation of the trachea or length of hospital stay.
Induction and Maintenance of Anesthesia
Midazolam is the benzodiazepine of choice for induction of anesthesia. Numerous factors influence the rapidity of action of midazolam and the other benzodiazepines when used for induction of general anesthesia, including dose, speed of injection, degree of premedication, age, ASA physical status, and concurrent anesthetic drugs. The usual induction dose of midazolam is 0.1 to 0.2 mg/kg in premedicated patients, and up to 0.3 mg/kg in unpremedicated patients. The onset of anesthesia is within 30 to 60 seconds. The half-time of equilibrium between the plasma concentration and the EEG effects is about 2 to 3 minutes.
Elderly patients require smaller doses of midazolam than younger patients ( Fig. 23.11 ).

When midazolam is combined with other anesthetic drugs (coinduction), often a synergistic interaction occurs, similar to that seen with propofol. This synergy is observed when midazolam is used with opioids or other hypnotics, similar to propofol in combination with opioids ( Fig 23.12 ).

Emergence time is related to the dose of midazolam and to the dose of adjuvant anesthetic drugs.
Benzodiazepines lack analgesic properties and must be used with other anesthetic drugs to provide sufficient analgesia; however, as maintenance anesthetic drugs during general anesthesia, benzodiazepines provide hypnosis and amnesia. The amnesic period after an anesthetic dose is about 1 to 2 hours.
A plasma level of more than 50 to 100 ng/mL occurs when used with adjuvant opioids (e.g., fentanyl) or inhaled anesthetics (e.g., nitrous oxide, volatile anesthetics) by a bolus initial dose of 0.05 to 0.15 mg/kg and a continuous infusion of 0.25 to 1 μg/kg/min. This plasma level is sufficient to keep the patient asleep and amnesic but arousable at the end of surgery. Smaller infusion doses may be required in some patients or in combination with opioids. Midazolam, diazepam, and lorazepam accumulate in the blood after repeated bolus administrations or with continuous infusion. If the benzodiazepines do accumulate with repeated administration, prolonged arousal time can be anticipated. This is less of a problem with midazolam than with diazepam and lorazepam because of the shorter context-sensitive half-time and greater clearance of midazolam. Remimazolam might be a good alternative; it is rapidly metabolized and has a faster recovery profile than midazolam in sheep.
Nausea and Vomiting Prophylaxis
Numerous studies have highlighted the role that benzodiazepines, and specifically midazolam, may play in the prevention of PONV. A recent meta-analysis on the effect of intravenous midazolam on PONV concludes a significant decrease in overall PONV and rescue antiemetic drug. Jung and colleagues found that in women undergoing middle ear surgery, IV midazolam, 0.075 mg/kg after induction of anesthesia, reduced the incidence of PONV and the need for rescue antiemetics with no difference from placebo in pain intensity or drowsiness. Furthermore, the combination of midazolam with dexamethasone proved more effective in preventing PONV than midazolam alone. The incidence of PONV after minor gynecologic or urologic surgery was the same when comparing IV ondansetron, 4 mg, and IV midazolam, 2 mg.
In children, IV midazolam, 0.05 mg/kg, reduced PONV significantly after pediatric (4-12 years old) strabismus surgery compared with placebo or IV dexamethasone 0.5 mg/kg. No child vomited with midazolam alone or with the midazolam dexamethasone combination.
Finally, in a 2010 three-arm, placebo-controlled, double-blind clinical trial, Fuji and associates compared midazolam 0.050 mg/kg with 0.075 mg/kg in patients undergoing laparoscopic gynecologic surgery regarding PONV prophylaxis. The two doses of midazolam were not significantly different with regard to PONV (30% vs. 27% of patients experienced PONV) and both proved better than placebo (67%).
Side Effects and Contraindications
Benzodiazepines have limited allergenic effects and do not suppress the adrenal gland. The most significant side effect with midazolam is respiratory depression. The major side effects of lorazepam and diazepam in addition to respiratory depression are venous irritation and thrombophlebitis, problems related to aqueous insolubility and requisite solvents. When used as sedative or for induction and maintenance of anesthesia, benzodiazepines may produce an undesirable degree or prolonged interval of postoperative amnesia, sedation, and, rarely, respiratory depression. These residual effects can be reversed with flumazenil.
Flumazenil
Flumazenil (Anexate, Romazicon) is the first benzodiazepine antagonist approved for clinical use. It is a benzodiazepine receptor ligand with high affinity, great specificity, and by definition minimal intrinsic effect. Flumazenil, similar to the agonists it replaces at the benzodiazepine receptor, interacts with the receptor in a concentration-dependent manner. It is a competitive antagonist at the benzodiazepine receptor and produces antagonism that is reversible and surmountable. In humans, flumazenil has minimal agonist activity, which means that its benzodiazepine receptor agonist effects are very weak, significantly less than those of clinical agonists. Flumazenil, similar to all competitive antagonists at receptors, does not displace the agonist, but rather occupies the receptor when an agonist dissociates from the receptor. The half-time (or half-life) of a receptor-ligand bond is a few milliseconds to a few seconds, and new ligand receptor bonds are immediately formed. This dynamic situation accounts for the ability of either an agonist or an antagonist to readily occupy the receptor. The ratio of agonist to total receptors produces the effects of the agonist drug, but the antagonist can alter this ratio, depending on its concentration and dissociation constant. Flumazenil, which is an avid (high-affinity) ligand, replaces a relatively weak agonist, such as diazepam, as long as it is given in sufficient dose. Flumazenil is rapidly metabolically cleared, however, and the proportion of receptors occupied by the agonist then increases again, and the potential for rebound sedation and respiratory suppression exists ( Fig. 23.13 ). This situation is less likely to occur when flumazenil is used to reverse midazolam, which has a more rapid clearance than other benzodiazepine agonists. Another important finding is that in the presence of extremely large doses of agonist (e.g., when a mistake in dosing has occurred, or suicide by means of an overdose is attempted), a small dose of flumazenil attenuates the deep CNS depression (loss of consciousness, respiratory depression) by reducing the fractional receptor occupancy by the agonist without decreasing the agonist effects that occur at low fractional receptor occupancy (drowsiness, amnesia).

Conversely, large doses of flumazenil in the presence of small doses of agonist completely reverse all the agonist effects. Flumazenil can precipitate withdrawal symptoms in animals or in humans physically dependent on a benzodiazepine receptor agonist. However, this is not a problem when flumazenil is used to reverse clinical effects of benzodiazepine receptor agonists applied in the practice of anesthesia.
Physicochemical Characteristics
Flumazenil is similar to midazolam and other classic benzodiazepines except for the absence of the phenyl group, which is replaced by a carbonyl group (see Fig. 23.8 ). It forms a colorless, crystalline powder; has a dissociation constant of 1.7; and has weak but sufficient water solubility to permit its preparation in aqueous solution. Its octanol/aqueous buffer (pH 7.4) partition coefficient is 14, showing moderate lipid solubility at pH 7.4.
Pharmacokinetics
Flumazenil, similar to the other benzodiazepines, is completely (99%) metabolized in the liver; is rapidly cleared from the plasma; and has three known metabolites: N -desmethylflumazenil, N -desmethylflumazenil acid, and flumazenil acid. The metabolic end product, that is, flumazenil acid, has no pharmacologic activity. The major metabolites identified in urine are the de-ethylated free acid and its glucuronide conjugate. Flumazenil is a short-lived compound. Box 23.2 includes a summary of its pharmacokinetics, which are described in various clinical settings. The volume of distribution is high and extravascular distribution is rapid.
Reversal of benzodiazepines | 0.2 mg repeated up to 3 mg |
Diagnosis in coma | 0.5 mg repeated up to 1 mg |
∗ The dose required to reverse each benzodiazepine (BZD) depends on residual BZD and the particular BZD (i.e., higher doses are required for more potent BZDs) (see text).
† The degree of reversal should be titrated by repeating 0.2-mg increments every 1 to 2 min until the desired level of reversal is achieved.
Compared with most benzodiazepine receptor agonists, flumazenil has a very rapid clearance and short elimination half-life. Only remimazolam has a more rapid clearance and shorter half-life. The plasma half-life of flumazenil is about 1 hour—it is the shortest lived of all benzodiazepines used in anesthetic practice. The rapid blood clearance of flumazenil approaches hepatic blood flow, a finding that indicates that liver clearance partially depends on hepatic blood flow. Compared with other benzodiazepines, flumazenil has a high proportion of unbound drug; plasma protein binding is about 40%. The potential exists for the antagonist to be cleared, leaving sufficient concentrations of agonist at the receptor site to cause resedation. To maintain a constant therapeutic blood level over a prolonged time, either repeated administration or a continuous infusion is required. An infusion rate of 30 to 60 μg/min (0.5-1 μg/kg/min) has been used for this purpose.
Pharmacodynamics
When given in the absence of a benzodiazepine receptor agonist, flumazenil has little discernible CNS effect. When given to healthy subjects and patients in clinically relevant doses, flumazenil has no effect on the EEG or cerebral metabolism. Flumazenil is free of anticonvulsant properties, and reverses the anticonvulsant properties of benzodiazepines in local anesthetic-induced seizures. When administered to patients who have benzodiazepine-induced CNS depression, flumazenil produces rapid and dependable reversal of unconsciousness, respiratory depression, sedation, amnesia, and psychomotor dysfunction. Flumazenil can be given before, during, or after the agonist to block or reverse the CNS effects of the agonist.
Flumazenil has successfully reversed the effects of benzodiazepines like midazolam, diazepam, lorazepam, and flunitrazepam. It has also been used successfully to reverse the effects of chloralhydrate and cannabis intoxication in children, carbamazepine and alcohol overdose, and antihistamines overdose. The onset is rapid, with peak effect occurring in 1 to 3 minutes, which coincides with the detection of C-flumazenil in the human brain. Flumazenil reverses the agonist by replacing it at the benzodiazepine receptor, and its onset and duration are governed by the law of mass action. When flumazenil is given in the presence of agonists, there are significant respiratory effects because it reverses respiratory depression caused by the agonists (e.g., when given to volunteers made apneic with midazolam). For example, the reversal of midazolam-induced (0.13 mg/kg) respiratory depression with flumazenil (1 mg) lasts 3 to 30 minutes. Other agonists and other doses would have different durations of antagonism of respiratory depression.
Incremental doses up to 3 mg intravenously in patients with ischemic heart disease had no significant effect on cardiovascular variables. Administration of flumazenil to patients given agonists is remarkably free of cardiovascular effects, in contrast to the experience of opioid reversal with naloxone. Although flumazenil does reverse sedation, it does not increase blood concentrations of catecholamines. However, catecholamine levels may increase when arousal is more rapid after flumazenil. The reversal of sedation by midazolam with flumazenil also restores the attenuated cardiac baroreflex function.
In healthy subjects, flumazenil did not alter intraocular pressure but reversed the decrease in intraocular pressure observed after administration of midazolam (Romazicon package insert; www.fda.gov ).
Uses and Doses
Application of a benzodiazepine antagonist (see Box 23.2 ) includes the diagnostic and therapeutic reversal of benzodiazepine receptor agonists. For diagnostic use in suspected benzodiazepine overdose, flumazenil may be given in incremental IV doses of 0.2 to 0.5 mg up to 3 mg. More commonly in anesthesia, flumazenil is used to reverse the residual sedation of a patient after administration of a benzodiazepine for premedication of a short surgical procedure, conscious sedation, or for general anesthesia. Flumazenil reliably reverses the sedation, respiratory depression, and amnesia caused by benzodiazepines. There are differential reversal effects on the different agonist actions. Flumazenil tends to reverse the hypnotic and respiratory effects more than the amnesic effects of the benzodiazepine agonist.
The dose varies with the particular benzodiazepine being reversed, and the duration of reversal depends on the kinetics of the agonist and of flumazenil. Surveillance is recommended if a long-lasting benzodiazepine is reversed with a single administration of flumazenil because of the relatively short-lived effect. If a patient shows no signs of recurrent sedation within 2 hours after a 1-mg reversal dose of flumazenil, serious recurrent sedation at a later time is unlikely. Flumazenil may be administered by continuous infusion to prevent recurrent sedation by longer lasting benzodiazepine receptor agonists. The pharmacokinetic profile of flumazenil is unaltered in the presence of benzodiazepine agonists (diazepam, flunitrazepam, lormetazepam, midazolam) and vice versa.
Side Effects and Contraindications
Flumazenil has been given in large oral and IV doses with remarkably few toxic reactions. It is free of local or tissue irritant properties, and there are no known organotoxicities. Similar to all benzodiazepines, it apparently has a high safety margin, probably higher than the safety margins of the agonists, because it does not produce prominent CNS depression. In patients using large doses of benzodiazepines over several weeks or longer, the administration of flumazenil may elicit symptoms of benzodiazepine withdrawal, including seizures.
Phencyclidines (Ketamine)
History
Ketamine (Ketalar) was synthesized in 1962 by Stevens and was first used in humans in 1965 by Corssen and Domino. Ketamine was released for clinical use in 1970 and is still used in various clinical settings. It produces dissociative anesthesia rather than generalized depression of the CNS through antagonistic actions at the phencyclidine (PCP) site of the N-methyl-D-aspartate receptor (NMDAR). Ketamine is a racemic mixture of the isomers R(-)-ketamine and S(+)-ketamine. It usually does not depress the cardiovascular and respiratory systems, but it does possess some of the adverse psychological effects found with the other phencyclidines. The S(+)-isomer (Ketanest) is 3 to 4 times more potent as an analgesic with a faster clearance and recovery and with fewer psychomimetic side effects. Still, S-ketamine produces—besides analgesia—psychotropic effects, cognitive impairment, memory impairment, and a reduced reaction time. Interest in ketamine has increased more recently because of its effects on hyperalgesia and opiate tolerance, use in chronic pain states, potential neuroprotective effects, increasing popularity of total IV anesthesia, and the availability in some countries of S(+) ketamine. Lastly, ketamine receives growing interest because of its antidepressant effects.
Physicochemical Characteristics
Ketamine ( Fig. 23.14 ) has a molecular weight of 238 kD, is partially water soluble, and forms a white crystalline salt with a p K a of 7.5. It has a lipid solubility 5 to 10 times that of thiopental. Ketamine is only 12% bound to proteins. Its bioavailability is 93% after parenteral administration but only 20% after oral use, due to its high first-pass metabolism.

Pharmacokinetics
Ketamine is metabolized by hepatic microsomal enzymes. The major pathway involves N -demethylation to form norketamine (metabolite I), which is then hydroxylated to hydroxynorketamine, which is further conjugated to water-soluble glucuronide derivates and excreted in the urine. The activity of the principal metabolites of ketamine has not been well studied, but norketamine (metabolite I) has significantly less (20%-30%) activity than the parent compound. More recent modeling of norketamine suggests that it contributes in prolonging the analgesia provided by either a bolus or infusion of ketamine, although this conclusion is being questioned. In contrast to previous reports, S-norketamine may have a negative contribution to S-ketamine-induced analgesia but shows absence of contribution to the cognitive impairment. This may explain the observation of ketamine-related excitatory phenomena (such as hyperalgesia and allodynia) upon the termination of ketamine infusions.
Ketamine’s pharmacokinetics have been examined after bolus administration of anesthetizing doses (2-2.5 mg/kg IV), after a subanesthetic dose (0.25 mg/kg IV), and after continuous infusion (steady-state plasma level 2000 ng/mL).
Regardless of the dose, ketamine plasma disappearance can be described by a two-compartment model. Table 23.1 contains the pharmacokinetic values from bolus administration studies. Of note is the rapid distribution reflected in the relatively brief distribution half-life of 11 to 16 minutes ( Fig. 23.15 ). The high lipid solubility of ketamine is reflected in its large volume of distribution of nearly 3 L/kg. Clearance also is high, 890 to 1227 mL/min, which accounts for the short elimination half-life of 2 to 3 hours. The mean total body clearance (1.4 L/min) is approximately equal to liver blood flow. Low-dose alfentanil increases the volume of distribution and clearance of ketamine. In addition, alfentanil increases the distribution of ketamine into the brain. The pharmacokinetic model of Clements provided the best accuracy when used to administer low-dose ketamine to volunteers using a target-controlled infusion device. The pharmacokinetics of the two isomers is different. S(+) ketamine has a larger elimination clearance and larger volume of distribution than R(−) ketamine. When the pharmacokinetics of S(+) ketamine were tested in a target-controlled infusion device for procedures of 1 hour and in combination with propofol, the authors found that the accuracy of the pharmacokinetic parameters was improved with a much smaller Vc ∗
∗ Vc (central compartment volume of a three compartment model).
(167 mL/kg). They also noted that ketamine clearance was not normally distributed, and this was not related to age. The S(+) enantiomer also seems to be more potent in suppressing the EEG than either R(−) or the racemic mixture. Ketamine is increasingly being given by alternative routes, especially orally and via an intranasal spray. Administration by either of these routes is subject to significant first-pass metabolism. The bioavailability via oral administration is 20% to 30%, and via the intranasal route is approximately 40% to 50%. In clinical and experimental studies hyperalgesic responses have been noted after the withdrawal of S (+)-ketamine. Furthermore, no delay between concentration and effect has been observed for any of the antinociceptive end points. This indicates an almost immediate passage of S(+)-ketamine across the blood-brain barrier and rapid receptor kinetics.
Pharmacodynamics
Effects on the Central Nervous System
Ketamine produces dose-related unconsciousness and analgesia. Ketamine acts at multiple receptors including the NMDAR, opioid receptors, and monoaminergic receptors. At high ketamine concentrations, sigma opioid receptors are also affected, muscarinic receptors are blocked, and GABAergic neurotransmission is facilitated. Its most important action is the inhibition of NMDAR-mediated glutamergic input to the GABAergic system leading to a changing excitatory activity in the cortex and limbic system that in the end results in unconsciousness. At the spinal cord level, ketamine has potent antinociceptive effects on NMDAR and inhibits acetylcholine release. The anesthetized state has been termed dissociative anesthesia because patients who receive ketamine alone appear to be in a cataleptic state, in contrast with other states of anesthesia that resemble normal sleep. Patients anesthetized with ketamine have profound analgesia, but keep their eyes open and maintain many reflexes. Corneal, cough, and swallow reflexes all may be present, but should not be assumed to be protective. There is no recall of surgery or anesthesia, but amnesia is not as prominent with ketamine as with the benzodiazepines. Because ketamine has a low molecular weight, a p K a near the physiologic pH, and relatively high lipid solubility, it crosses the blood-brain barrier rapidly and has an onset of action within 30 to 60 seconds of administration. The maximal effect occurs in about 1 minute.
After ketamine administration, pupils dilate moderately, and nystagmus occurs. Lacrimation and salivation are common, as is increased skeletal muscle tone, often with coordinated but seemingly purposeless movements of the arms, legs, trunk, and head. Although there is great interindividual variability, plasma levels of 0.6 to 2 μg/mL are considered the minimum concentrations for general anesthesia; children may require slightly higher plasma levels (0.8-4 μg/mL). The duration of ketamine anesthesia after a single IV administration of a general anesthetic dose (2 mg/kg) is 10 to 15 minutes (see Fig. 23.15 ), and full orientation to person, place, and time occurs within 15 to 30 minutes. The S(+) enantiomer enables quicker recovery (by a couple of minutes) than the racemic mixture. This is due to the smaller dose necessary to produce an equianesthetic effect and to the 10% faster hepatic biotransformation. Because there is a good correlation between blood concentration of ketamine and CNS effect, the relatively short duration of action of ketamine is probably due to its redistribution from the brain and blood to the other tissues in the body.
Concomitant administration of benzodiazepines, which is a common practice, may prolong the effect of ketamine. When used in combination with a benzodiazepine, the S(+) enantiomer was no different in terms of awareness at 30 minutes, but it was significantly better at 120 minutes than the racemic mixture. Analgesia occurs at considerably lower blood concentrations than loss of consciousness.
Ketamine provides important postoperative analgesia. The plasma level at which pain thresholds are elevated is 0.1 μg/mL or greater. This means there is a considerable period of postoperative analgesia after ketamine general anesthesia, and subanesthetic doses can consequently be used to produce analgesia. Ketamine inhibits nociceptive central hypersensitization. Ketamine also attenuates acute tolerance after opiate administration. The NMDAR plays an important role in the induction of hyperalgesia and antinociceptive tolerance as induced by opioids. Preventive ketamine administration may thus prevent central sensitization and long-lasting enhancement in pain sensitivity as induced by opioids. Ketamine, just as other NMDAR antagonists, may thus prevent opioid-induced hyperalgesia. The primary site of CNS action of ketamine seems to be the thalamoneocortical projection system. The drug selectively depresses neuronal function in parts of the cortex (especially association areas) and thalamus, while stimulating parts of the limbic system, including the hippocampus. This process creates what is termed a functional disorganization of nonspecific pathways in midbrain and thalamic areas. Ketamine acts via antagonism of the excitatory glutamatergic NMDA receptor. The NMDA receptor has a high expression in the temporal cortex, hippocampus, basal ganglia, cerebellum, and brainstem, all regions significantly affected by ketamine. There is also evidence that ketamine depresses transmission of impulses in the medial medullary reticular formation, which is important for transmission of the affective-emotional components of nociception from the spinal cord to higher brain centers. In volunteers experiencing heat pain, functional magnetic resonance imaging (MRI) studies showed ketamine produced a dose-dependent effect on pain processing by decreasing activation of the secondary somatosensory cortex (S2), insula, and anterior cingulate cortex. Ketamine occupies opiate receptors in the brain and spinal cord, and this property could account for some of the analgesic effects. The S(+) enantiomer has some opioid μ-receptor activity, accounting for part of its analgesic effect. NMDA receptor interaction may mediate the general anesthetic effects and some analgesic actions of ketamine. The spinal cord analgesic effect of ketamine is postulated to be due to inhibition of dorsal horn wide dynamic range neuronal activity. In a resting state fMRI study, low-dose ketamine induced connectivity changes in brain areas involved in motor function, psychedelic effects, and pain processing. Ketamine’s analgesic effect may arise from multiple pathways; a decreased connectivity in regions of the pain matrix responsible for the perception of pain (pain sensing) and the affective processing of pain. In addition, ketamine affected connectivity in brain areas involved in endogenous pain inhibition.
Although some drugs have been used to antagonize ketamine, no specific receptor antagonist reverses all the CNS effects of ketamine.
Ketamine increases cerebral metabolism, CBF, and ICP. Because of its excitatory CNS effects, which can be detected by generalized EEG development of theta wave activity and by petit mal seizure-like activity in the hippocampus, ketamine increases CMRO 2 . There is an increase in CBF, which appears higher than the increase in CMRO 2 would mandate. With the increase in CBF and the generalized increase in sympathetic nervous system response, there is an increase in ICP after ketamine. The increase in CMRO 2 and CBF can be blocked by the use of thiopental or diazepam. Cerebrovascular responsiveness to carbon dioxide seems to be preserved with ketamine; reducing PaCO 2 attenuates the increase in ICP after ketamine.
S(+) ketamine may influence the expression of apoptosis-regulating proteins in rat brains 4 hours after cerebral ischemia/reperfusion. The neuroprotection observed with ketamine may involve antiapoptotic mechanisms in addition to reducing necrotic cell death.
In contrast, ketamine and other anesthetics like propofol and volatile anesthetics, accentuate apoptosis in the brain of newborn animals and cause changes in the morphology of dendritic spines. This finding has sparked controversy over the use of ketamine in neonates. An editorial in the journal Anesthesiology and the Anesthetic and Life Support Drugs Advisory Committee of the FDA cautioned changing clinical practice based on present available data.
Ketamine, similar to other phencyclidines, produces undesirable psychological reactions, which occur during awakening from ketamine anesthesia and are termed emergence reactions . The common manifestations of these reactions, which vary in severity and classification, are vivid dreaming, extracorporeal experiences (sense of floating out of body), and illusions (misinterpretation of a real, external sensory experience). These incidents of dreaming and illusion are often associated with excitement, confusion, euphoria, and fear. They occur in the first hour of emergence and usually abate within 1 to several hours. The psychic emergence reactions occur secondary to ketamine-induced depression of auditory and visual relay nuclei, leading to misperception or misinterpretation of auditory and visual stimuli. The incidence of the psychic emergence reactions ranges from 3% to 100%. A clinically relevant range is probably 10% to 30% of adult patients who receive ketamine as a sole or major part of the anesthetic technique. Factors that affect the incidence of emergence reactions are age, dose, gender, psychological susceptibility, and concurrent drugs. Pediatric patients do not report as high an incidence of unpleasant emergence reactions as do adult patients; men also report a less frequent incidence compared with women. Larger doses and rapid administration of large doses seem to predispose patients to a frequent incidence of adverse effects. Finally, certain personality types seem prone to the development of emergence reactions. Patients who score high in psychotism on the Eysenck Personality Inventory are prone to develop emergence reactions, and individuals who commonly dream at home are more likely to have postoperative dreams in the hospital after ketamine. While numerous drugs have been used to reduce the incidence and severity of postoperative reactions to ketamine, the benzodiazepines seem to be the most effective group of drugs. Next to the undesirable psychological reactions, increasingly ketamine is described for its antidepressant effects. The dose often used for this indication is 0.5 mg/kg, given as a 40-minute infusion. This often results in a dramatic mood change within a day, often lasting for 3 to 12 days. A maintenance dose every 2 to 4 days may lengthen this effect. The precise mechanism of action of the antidepressant effects of ketamine remain unknown.
Effects on the Respiratory System
Ketamine has minimal effects on the central respiratory drive as reflected by an unaltered response to carbon dioxide. There can be a transient (1-3 minutes) decrease in minute ventilation after the bolus administration of an induction dose of ketamine (2 mg/kg intravenously). Unusually large doses can produce apnea, but this is seldom seen. In a μ-opioid knockout mouse model, though, at supraspinal sites S(+) ketamine interacts with the μ-opioid receptor system. This interaction contributes significantly to S(+) ketamine-induced respiratory depression and supraspinal antinociception. With the use of adjuvant sedatives or anesthetic drugs, respiratory depression may become clinically significant. Ketamine depresses ventilatory control in children especially with bolus doses. Ketamine is a bronchial smooth muscle relaxant. When it is given to patients with reactive airway disease and bronchospasm, pulmonary compliance is improved.
Ketamine is as effective as halothane or enflurane in preventing experimentally induced bronchospasm. The mechanism for this effect is probably a result of the sympathomimetic response to ketamine, but there are isolated bronchial smooth muscle studies showing that ketamine can directly antagonize the spasmogenic effects of carbachol and histamine. Owing to its bronchodilating effect, administration of ketamine can treat status asthmaticus unresponsive to conventional therapy. A potential respiratory problem, especially in children, is the increased salivation that follows ketamine administration, which can be modulated by an anticholinergic drug such as atropine or glycopyrolate.
Effects on the Cardiovascular System
Ketamine increases arterial blood pressure, heart rate, and cardiac output in a biphasic manner. It produces a direct cardiodepressive, negative inotropic effect next to an indirect stimulatory effect due to activation of the sympathetic system. Ketamine causes the systemic release of catecholamines, inhibition of the vagal nerve, inhibition of norepinephrine reuptake at peripheral nerves and non-neuronal tissues such as the myocardium, and norepinephrine release from sympathetic ganglia. Cardiodepression precedes stimulation after large-dose ketamine administration or occurs after repeated administrations when presynaptic catecholamine stores become depleted. Cardiovascular stimulation already occurs after small-dose ketamine infusion and is characterized by tachycardia, systemic and pulmonary hypertension, increases in cardiac output, and myocardial oxygen consumption. Whereas the cardiovascular stimulatory effects of ketamine generally are dominant, after termination of S-ketamine infusion, cardiovascular depression may become evident as cardiac output may decrease below pre-infusion values. The cardiovascular stimulatory effects of S(+) ketamine are characterized by an increase in the cardiac output of 1 L/min in the presence of 243 ng/ml S(+) ketamine. The cardiovascular stimulatory effect of S(+) ketamine is induced very rapidly with a half-life for onset/offset of the effect of ketamine on cardiac output of 1 to 2 minutes. The increase in hemodynamic variables is associated with increased work and myocardial oxygen consumption. The healthy heart increases oxygen supply by increased cardiac output and decreased coronary vascular resistance, so that coronary blood flow is appropriate for the increased oxygen consumption. In patients with congenital heart disease, there are no significant changes in shunt directions or fraction, or systemic oxygenation after ketamine induction of anesthesia. In patients who have increased pulmonary artery pressure (as with mitral valvular and some congenital lesions), ketamine causes a more pronounced increase in pulmonary than systemic vascular resistance. Ketamine injected directly into the CNS produces an immediate sympathetic nervous system hemodynamic response. Ketamine also causes the sympathoneuronal release of norepinephrine, which can be detected in venous blood. Blockade of this effect is possible with barbiturates, benzodiazepines, and droperidol. The centrally mediated sympathetic responses to ketamine usually override the direct depressant effects of ketamine. Some peripheral nervous system actions of ketamine play an undetermined role in the hemodynamic effects of the drug. Ketamine inhibits intraneuronal uptake of catecholamines in a cocaine-like effect and inhibits extraneuronal norepinephrine uptake.
Stimulation of the cardiovascular system is not always desirable, and certain pharmacologic methods have been used to block the ketamine-induced tachycardia and systemic hypertension. Probably the most fruitful approach has been prior administration of benzodiazepines. Modest doses of diazepam, flunitrazepam, and midazolam all attenuate the hemodynamic effects of ketamine. It also is possible to decrease the tachycardia and hypertension caused by ketamine by using a continuous infusion technique with or without a benzodiazepine. Inhalation anesthetics and propofol blunt the hemodynamic effect of ketamine.
Uses
The many unique features of ketamine pharmacology, especially its propensity to produce unwanted emergence reactions in 10% to 20% of patients, have limited its use for routine anesthesia. Nevertheless, ketamine has an important niche in the practice of anesthesiology when its unique sympathomimetic activity and bronchodilating capabilities are indicated during induction of anesthesia. It is used for premedication, sedation, induction, and maintenance of general anesthesia. There has been increased interest in the use of ketamine in small doses for preventive analgesia, for the treatment or prevention of opiate tolerance and hyperalgesia, and in treatment of acute and chronic pain.
Induction and Maintenance of Anesthesia
The cardiovascular stimulatory effects make ketamine a desirable drug for the induction of anesthesia in unstable cardiovascular patients suffering from hypovolemia, hemorrhagic shock, or cardiovascular depression in sepsis. Ketamine bronchodilation and profound analgesia allowing the use of high oxygen concentrations make ketamine an excellent choice for induction of anesthesia in patients with reactive airway disease. Trauma patients with extensive blood loss are typical candidates for rapid-sequence anesthesia induction with ketamine. Patients with septic shock also may benefit from ketamine. The intrinsic myocardial depressant effect of ketamine may manifest in this situation if trauma or sepsis has caused depletion of catecholamine stores before the patient’s arrival in the operating room. Use of ketamine in these patients does not obviate the need for appropriate preoperative preparation, including restoration of intravascular blood volume. Other cardiac diseases that can be well managed with ketamine anesthesia are cardiac tamponade and restrictive pericarditis. The finding that ketamine preserves heart rate and right atrial pressure through its sympathetic stimulating effects makes ketamine an excellent anesthetic induction and maintenance drug in this setting. Ketamine also is often used in patients with congenital heart disease, especially patients in whom the propensity for right-to-left shunting exists. Ketamine has been successfully used in a patient susceptible to malignant hyperthermia. Ketamine combined with propofol or midazolam can be given by continuous infusion to produce satisfactory cardiac anesthesia for patients with valvular and ischemic heart disease. The combination of a benzodiazepine or of a benzodiazepine plus sufentanil with ketamine attenuates or eliminates the unwanted tachycardia and hypertension and postoperative psychological derangements. With this technique, there are minimal hemodynamic perturbations, profound analgesia, dependable amnesia, and an uneventful convalescence. The use of propofol plus small-dose ketamine also has gained increasing popularity as a total IV anesthesia technique for patients undergoing noncardiac surgery. The advantages of this combination are maintenance of stable hemodynamics and minimal ventilatory depression when allowing spontaneous ventilation.
Pain Management
Postoperative pain is a major concern of many patients and inadequately treated in as many as 30% to 50% of all postoperative patients. Multimodal analgesia combining various analgesic agents that act through different pathways is the way to manage postoperative pain. Ketamine is increasingly used as one of the constituents of this multimodal analgesia therapy. Over the years the ketamine dose used for perioperative analgesia has gradually been decreasing. Ketamine administered in small doses decreases postoperative analgesic consumption by 33%. Several meta-analyses of the use of small-dose ketamine (20 to 60 mg) perioperatively have been performed. These meta-analyses showed an overall decrease in opiate use or improved analgesia and a decrease in opiate-induced side effects, especially PONV. Side effects, especially psychomimetic effects, were minimal, especially if a benzodiazepine also was administered.
The epidural/caudal administration of ketamine (0.5 to 1 mg/kg) is effective. Although the efficacy of these doses of ketamine seems to be established, the safety of this technique has not yet received regulatory approval. The preservative of racemic mixture is potentially neurotoxic, whereas studies to date indicate preservative-free S(+) ketamine may be safe. Epidural preservative-free S(+) ketamine has been shown to be safe and of value in adjunct to corticosteroids in patients for the treatment of chronic low back pain secondary to radiculopathy. The favorable hemodynamic effects and conservation of respiration makes intravenously and even intranasally administered ketamine useful for analgesia after extremity fractures.
The action of ketamine on opiate tolerance and hyperalgesia combined with its direct analgesic activity has led to its use in chronic pain states. Ketamine may be effective in the treatment of cancer pain, chronic peripheral and central neuropathic pain, phantom and ischemic limb pain, fibromyalgia, complex regional pain syndrome, visceral pain, and migraine. Multiple open-label studies conclude positively on the analgesic properties of ketamine in cancer pain. Randomized controlled trials, though, so far could not prove a clinical benefit of ketamine for this indication. Thus, while ketamine is effective for relief of postoperative pain, causing reduced opioid consumption, ketamine for most other indications appears to have limited efficacy and results in no beneficial effects.
Sedation
Often, ketamine is combined with premedication of a barbiturate or benzodiazepine and an antisialagogue (e.g., glycopyrrolate) to facilitate management. The premedications reduce the dose requirement for ketamine, and the antisialagogue reduces the sometimes troublesome salivation. In adults and children, ketamine can be used as a supplement or an adjunct to regional anesthesia, extending the usefulness of the primary (local anesthetic) form of anesthesia. Also, in the emergency department ketamine is increasingly used for short painful procedures. The dose used then is between 0.1 to 0.6 mg/kg. As previously described, ketamine also may be considered for sedation of patients in a critical care unit because of its combined sedative and analgesic properties and favorable effects on hemodynamics. Ketamine can even be used safely in head injury patients when they are adequately ventilated.
Ketamine is particularly suitable for sedation of pediatric patients undergoing procedures outside of the operating room. Pediatric patients have fewer adverse emergence reactions than adults, and this feature makes the use of ketamine in pediatric patients more versatile.
Doses and Routes of Administration
Ketamine has been administered intravenously, intramuscularly, transcutaneously, orally, nasally, and rectally, and as a preservative-free solution epidurally or intrathecally. Most clinical use involves the IV and intramuscular (IM) routes, by which the drug rapidly achieves therapeutic concentrations. The dose depends on the desired therapeutic effect and on the route of administration. Box 23.3 contains general recommended doses for the IV and IM administration of ketamine for various therapeutic goals. Intranasal administration has an onset closer to IV administration; an oral dose of 3 to 10 mg/kg generates a sedative effect in 20 to 45 minutes. For sedation, ketamine may be given in an IM dose of 2 to 4 mg/kg. It also has been administered orally in doses of 3 to 10 mg/kg, with 6 mg/kg providing optimal conditions in 20 to 25 minutes in one study and 10 mg/kg providing sedation in 87% of children within 45 minutes in another study.
Induction of general anesthesia ∗ | 0.5-2 mg/kg IV 4-6 mg/kg IM |
Maintenance of general anesthesia | 0.5-1 mg/kg IV with N 2 O 50% in O 2 15-45 μg/kg/min IV with N 2 O 50%-70% in O 2 |
30-90 μg/kg/min IV without N 2 O | |
Sedation and analgesia | 0.2-0.8 mg/kg IV over 2-3 min |
2-4 mg/kg IM | |
Preemptive or preventive analgesia | 0.15-0.25 mg/kg IV |
∗ Lower doses are used if adjuvant drugs such as midazolam or thiopental also are given. IM, Intramuscular; IV, intravenous; N 2 O, nitrous oxide.
Side Effects and Contraindications
Contraindications to ketamine relate to specific pharmacologic actions and patient diseases. In patients with increased ICP and breathing spontaneously, ketamine should be used with caution because it can increase ICP and has been reported to cause apnea. There is increasing clinical use of ketamine in emergency airway management in brain injury patients with or without other body injuries. In this setting, the current knowledge on safe management of increased ICP is continuing to grow.
In mechanically ventilated patients, ketamine retains the response of CBF to carbon dioxide, which makes it useful in head-injured patients because of its potential neuroprotective effect. Ketamine may be contraindicated in patients with an open eye injury or other ophthalmologic disorder, in which a ketamine induced increase in intraocular pressure would be detrimental. Because ketamine has a propensity to cause hypertension and tachycardia, with a commensurate increase in myocardial oxygen consumption, it may be contraindicated as the sole anesthetic in patients with ischemic heart disease. Likewise, it is unwise to give ketamine to patients with vascular aneurysms because of the possible sudden change in arterial blood pressure. Psychiatric disease, such as schizophrenia, and a history of adverse reaction to ketamine or one of its congeners also are contraindications. One also should consider carefully using ketamine when there is a possibility of postoperative delirium from other causes (e.g., delirium tremens, possibility of head trauma), and a ketamine-induced psychomimetic effect would confuse the differential diagnosis.
As mentioned earlier, ketamine or other NMDA receptor antagonists accentuate apoptosis in the newborn brain of animals, and the clinical implications of this are unknown. Finally, because ketamine’s preservative—chlorobutanol—is neurotoxic, this formulation of ketamine for subarachnoid or epidural administration is contraindicated. S(+) ketamine is available in a preservative-free solution. The FDA has not approved the use of intrathecal or epidural ketamine. Caudal ketamine has been used for perioperative analgesia in children and neonates with 0.5 mg/kg as the optimal dose. Caudal analgesia, by combinations of ketamine and a local anesthetic, prolongs analgesia from 2.26 to 5.3 hours and reduces the need for nonopioid analgesics.
Lastly, liver and renal toxicity occurs in the recreational abuse of ketamine. In addition, when ketamine is repeatedly administered in the treatment of chronic pain in patients with complex regional pain syndrome type 1 (CRPS), hepatotoxicity developed in patients that received two 100-hour infusions of S(+)-ketamine with a 16-day interval.
Etomidate
History
The first report on etomidate was published in 1965. Etomidate was introduced into clinical practice in 1972. The unique properties of etomidate include hemodynamic stability, minimal respiratory depression, cerebral protection, favorable toxicity profile, and pharmacokinetics enabling rapid recovery after either a single dose or a continuous infusion. In the 1970s, these beneficial properties led to widespread use of etomidate for induction, for maintenance of anesthesia, and for prolonged sedation in critically ill patients. The enthusiasm among clinicians for etomidate was tempered in the 1980s by reports that the drug can cause temporary inhibition of steroid synthesis after single doses and infusions. This effect, combined with other minor disadvantages (e.g., pain on injection, superficial thrombophlebitis, myoclonus, and a frequent incidence of nausea and vomiting), led to several editorials questioning the role of etomidate in modern anesthetic practice. Use of the drug decreased after those editorials, but its use has expanded again as a result of the rediscovery of etomidate’s beneficial physiologic profile and a widening use in emergency departments and intensive care departments, combined with a lack of novel reports describing clinically significant adrenocortical suppression after induction or brief duration infusions.
Physicochemical Characteristics
Etomidate is an imidazole derivative (R-(+)-pentylethyl-1H-imidazole-5 carboxylate sulfate). Its chemical structure is illustrated in Fig. 23.16 . Etomidate has a p K a of 4.2 and is hydrophobic at physiologic pH. To increase its solubility it is formulated as a 0.2% solution either in 35% propylene glycol (Amidate; Hospira Inc., Lafe Forest, IL) or in a lipid emulsion (Etomidate-Lipuro; B. Braun, Melsungen, Germany).

Pharmacokinetics
The pharmacokinetics of etomidate have been determined after single bolus doses and after continuous infusion. The time course of plasma disappearance after a 0.3 mg/kg bolus is shown in Fig. 23.17 . The pharmacokinetics of etomidate are best described by an open three-compartment model.

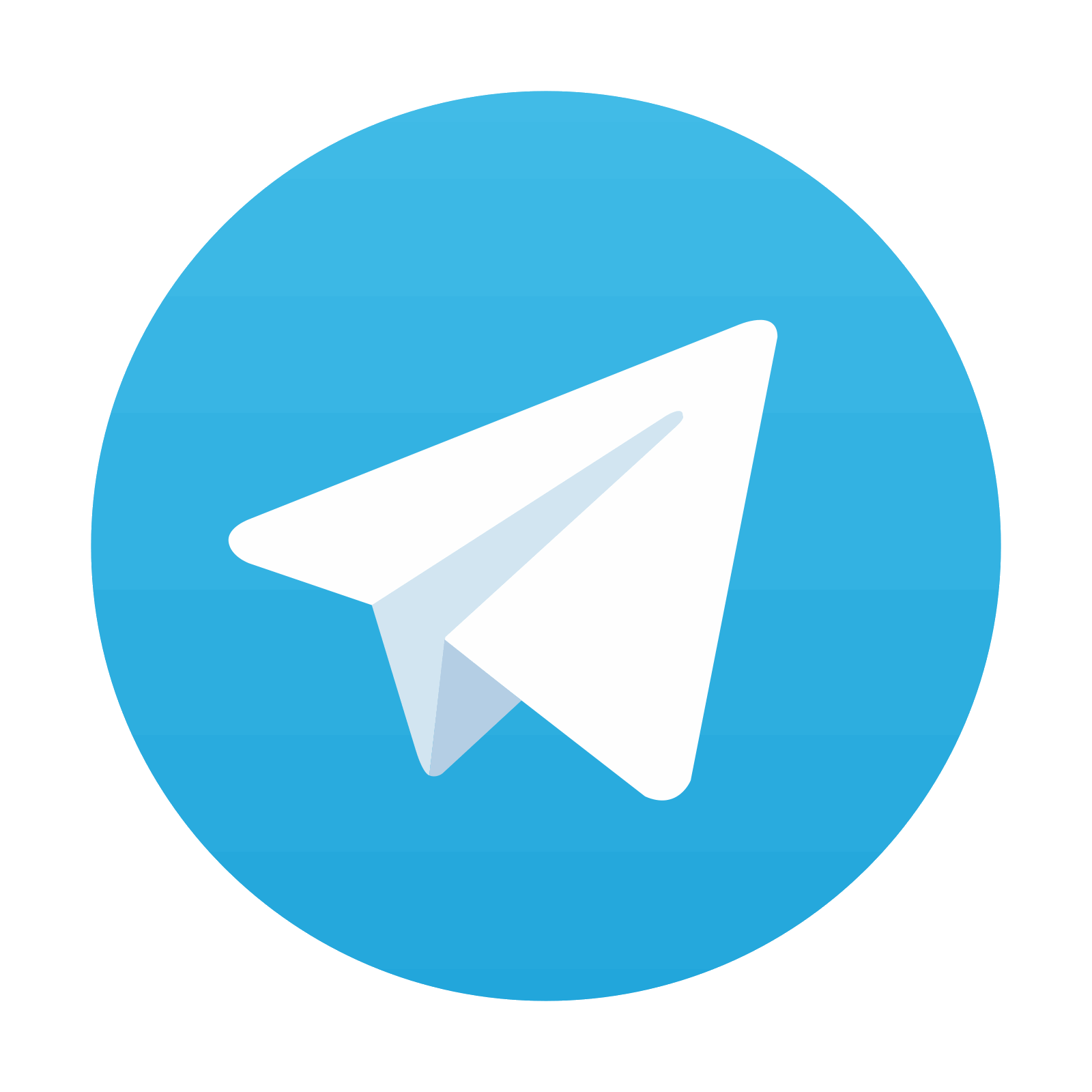
Stay updated, free articles. Join our Telegram channel
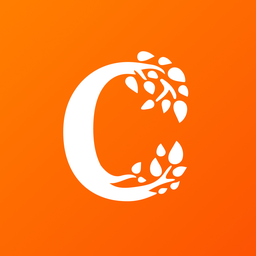
Full access? Get Clinical Tree
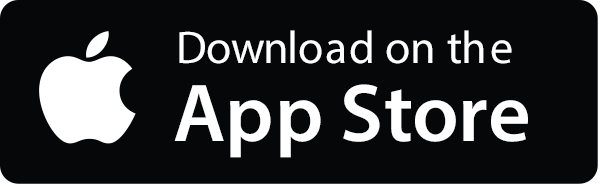
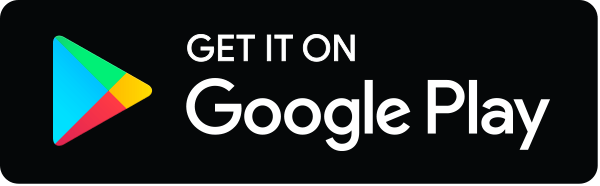
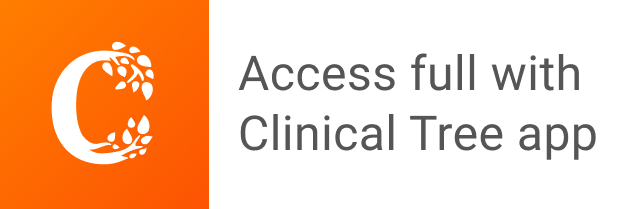