Halothane was synthesized in 1951 and introduced for clinical use in 1956. However, the tendency for alkane derivatives such as halothane to enhance the arrhythmogenic effects of epinephrine led to the search for new inhaled anesthetics derived from ethers. Methoxyflurane, a methyl ethyl ether, was the first such derivative. Methoxyflurane was introduced into clinical practice in 1960. Although methoxyflurane did not enhance the arrhythmogenic effects of epinephrine, its high solubility in blood and lipids resulted in a prolonged induction and slow recovery from anesthesia. More importantly, methoxyflurane caused hepatic toxicity. Extensive hepatic metabolism increased plasma concentrations of fluoride, which caused nephrotoxicity, especially with prolonged exposures to the anesthetic. Methoxyflurane has analgesic properties at concentrations far below those that induce anesthesia. Although its use was abandoned in the United States and Canada in the 1970s, it continues to be used in Australia for brief painful procedures and emergency transport.3 Enflurane, the next methyl ethyl ether derivative, was introduced for clinical use in 1973. This anesthetic, in contrast to halothane, does not enhance the arrhythmogenic effects of epinephrine or cause hepatotoxicity. Nevertheless, side effects were present, including metabolism to inorganic fluoride and stimulation of the central nervous system (CNS), lowering the seizure threshold. In search of a drug with fewer side effects, isoflurane, a structural isomer of enflurane, was introduced in 1981. This drug was resistant to metabolism, making organ toxicity unlikely after its administration.
Inhaled Anesthetics for the Present and Future
The search for even more pharmacologically “perfect” inhaled anesthetics did not end with the introduction and widespread use of isoflurane. The exclusion of all halogens except fluorine results in nonflammable liquids that are poorly lipid soluble and extremely resistant to metabolism. Desflurane, a totally fluorinated methyl ethyl ether, was introduced in 1992 and was followed in 1994 by the totally fluorinated methyl isopropyl ether, sevoflurane.4,5 The low solubility of these volatile anesthetics in blood facilitated rapid induction of anesthesia, precise control of end-tidal anesthetic concentrations during maintenance of anesthesia, and prompt recovery at the end of anesthesia independent of the duration of administration. The development, introduction, and rapid clinical acceptance of desflurane and sevoflurane reflects market forces (ambulatory surgery and the desire for rapid awakening possible with poorly soluble but potent anesthetics) more than an improved pharmacologic profile on various organ systems as compared with isoflurane. The challenge to the anesthesiologist is to exploit the pharmacokinetic advantages of these drugs while minimizing the risks (airway irritation, sympathetic nervous system stimulation, carbon monoxide production from interaction with carbon dioxide absorbent and complex vaporizer technology with desflurane, and compound A production from sevoflurane) and the increased expense associated with the manufacture and increased cost of administration of desflurane and sevoflurane.
Cost Considerations
Cost is an important consideration in the adoption of new drugs, including inhaled anesthetics. Factors that may influence the cost of a new inhaled anesthetics include (a) price (cost per milliliter of liquid); (b) inherent characteristics of the anesthetic, such as its vapor pressure (milliliter of vapor available per milliliter of liquid), potency, and solubility; and (c) fresh gas flow rate selected for delivery of the anesthetic.6 The costs of new inhaled anesthetics can be decreased by using low fresh gas flow rates. Less soluble anesthetics are more suitable for use with low gas flow rates because their poor solubility permits better control of the delivered concentration. Furthermore, there is less depletion of these anesthetics from the inspired gases so that fewer molecules need to be added to the returning rebreathed gases. This conservation offsets the decreased potency of a drug such as desflurane compared with isoflurane. For example, desflurane is one-fifth as potent as isoflurane, yet the amount of desflurane that must be delivered to sustain minimal alveolar concentration (MAC) is only slightly more than threefold the amount of isoflurane. Similarly, although MAC of sevoflurane is 74% greater than isoflurane, the amount of sevoflurane that must be delivered to sustain MAC is only 30% greater.
Current Clinically Useful Inhaled Anesthetics
Commonly administered inhaled anesthetics include the inorganic gas nitrous oxide and the volatile liquids isoflurane, desflurane, and sevoflurane (Table 4-1) (Fig. 4-2).4,5 Halothane and enflurane are administered infrequently but are included in the discussion of the comparative pharmacology of volatile anesthetics since halothane in particular has been studied extensively.4,5


Volatile liquids are administered as vapors after their vaporization in devices known as vaporizers. Diethyl ether and chloroform are still available, but mostly used only in veterinary medicine. Xenon is an inert gas with anesthetic properties, but its clinical use is hindered by its high cost.7
Nitrous Oxide
Nitrous oxide is a low-molecular-weight, odorless to sweet-smelling nonflammable gas of low potency and poor blood solubility (blood:gas partition coefficient 0.46) that is most commonly administered in combination with opioids or volatile anesthetics to produce general anesthesia. Although nitrous oxide is nonflammable, it will support combustion.8 Its poor blood solubility permits rapid achievement of an alveolar and brain partial pressure of the drug (Fig. 4-3). The analgesic effects of nitrous oxide are prominent but short lived, dissipating after about 20 minutes of use while sedative effects persist.9 Nitrous oxide causes minimal skeletal muscle relaxation. The speculated role of nitrous oxide in postoperative nausea and vomiting is controversial. Although much studied, the results of double-blind randomized trials have varied. A recent meta-analysis that included 30 published studies suggests that avoidance of nitrous oxide is associated with a lower risk of postoperative nausea and vomiting (RR = 0.80 [0.71–0.90]).10 Nitrous oxide has no effect on tissue PO2 measurements but does cause a small increase in the P50 (about 1.6 mm Hg).11 The benefits of nitrous oxide must be balanced against its possible adverse effects related to the high-volume absorption of nitrous oxide in gas-containing spaces, potential increase in the risk of postoperative nausea and vomiting, and its ability to inactivate vitamin B12.

Halothane
Halothane is a halogenated alkane derivative that exists as a clear, nonflammable liquid at room temperature. The vapor of this liquid has a sweet, nonpungent odor. An intermediate solubility in blood, combined with a high potency, permits intermediate onset and recovery from anesthesia using halothane alone or in combination with nitrous oxide or injected drugs such as opioids.
Halothane was developed on the basis of predictions that its halogenated structure would provide nonflammability, intermediate blood solubility, anesthetic potency, and molecular stability. Specifically, carbon-fluorine decreases flammability, and the trifluorocarbon contributes to molecular stability. The presence of a carbon-chlorine and carbon-bromine bond plus the retention of a hydrogen atom ensures anesthetic potency. Despite its chemical stability, halothane is susceptible to decomposition to hydrochloric acid, hydrobromic acid, chloride, bromide, and phosgene. For this reason, halothane is stored in amber-colored bottles, and thymol is added as a preservative to prevent spontaneous oxidative decomposition. Thymol that remains in vaporizers after vaporization of halothane can cause vaporizer turnstiles or temperature-compensating devices to malfunction.
Enflurane
Enflurane is a halogenated methyl ethyl ether that exists as a clear, nonflammable volatile liquid at room temperature and has a pungent, ethereal odor. Its intermediate solubility in blood combined with a high potency permits intermediate onset and recovery from anesthesia, using enflurane alone or in combination with nitrous oxide or injected drugs such as opioids. Enflurane decreases the threshold for seizures. Enflurane is oxidized in the liver to produce inorganic fluoride ions that can be nephrotoxic. It is primarily used for procedures in which a low threshold for seizure generation is desirable, such as electroconvulsive therapy.
Isoflurane
Isoflurane is a halogenated methyl ethyl ether that exists as a clear, nonflammable liquid at room temperature and has a pungent, ethereal odor. Its intermediate solubility in blood combined with a high potency permits intermediate onset and recovery from anesthesia using isoflurane alone or in combination with nitrous oxide or injected drugs such as opioids.
Although isoflurane is an isomer of enflurane, their manufacturing processes are not similar. The compounds used at the start of manufacturing are different, with 2,2,2-trifluoroethanol the starting compound for isoflurane and chlorotrifluoroethylene for enflurane. The subsequent purification of isoflurane by distillation is complex and expensive. Isoflurane is characterized by extreme physical stability, undergoing no detectable deterioration during 5 years of storage or on exposure to carbon dioxide absorbents or sunlight. The stability of isoflurane obviates the need to add preservatives such as thymol to the commercial preparation.
Desflurane
Desflurane is a fluorinated methyl ethyl ether that differs from isoflurane only by substitution of a fluorine atom for the chlorine atom found on the alpha-ethyl component of isoflurane. Fluorination rather than chlorination increases vapor pressure (decreases intermolecular attraction), enhances molecular stability, and decreases potency. Indeed, the vapor pressure of desflurane exceeds that of isoflurane by a factor of three such that desflurane would boil at normal operating room temperatures. A new vaporizer technology addressed this property, producing a regulated concentration by converting desflurane to a gas (heated and pressurized vaporizer that requires electrical power), which is then blended with diluent fresh gas flow. The only evidence of metabolism of desflurane is the presence of measurable concentrations of serum and urinary trifluoroacetate that are one-fifth to one-tenth those produced by the metabolism of isoflurane. The potency of desflurane as reflected by MAC is about fivefold less than isoflurane.
Unlike halothane and sevoflurane, desflurane is pungent, making it unlikely that inhalation induction of anesthesia would be feasible or pleasant for the patient. Indeed, the pungency of desflurane produces airway irritation and an appreciable incidence of salivation, breath-holding, coughing, or laryngospasm when >6% inspired desflurane is administered to an awake patient.4 Carbon monoxide results from degradation of desflurane by the strong base present in desiccated carbon dioxide absorbents. Desflurane produces the highest carbon monoxide concentrations, followed by enflurane and isoflurane, whereas amounts produced from halothane and sevoflurane are trivial.
Solubility characteristics (blood:gas partition coefficient 0.45) and potency (MAC 6.6%) permit rapid achievement of an alveolar partial pressure necessary for anesthesia followed by prompt awakening when desflurane is discontinued. It is this lower blood-gas solubility and more precise control over the delivery of anesthesia and more rapid recovery from anesthesia that distinguish desflurane (and sevoflurane) from earlier volatile anesthetics.
Sevoflurane
Sevoflurane is a fluorinated methyl isopropyl ether. The vapor pressure of sevoflurane resembles that of halothane and isoflurane, permitting delivery of this anesthetic via a conventional unheated vaporizer. The solubility of sevoflurane (blood:gas partition coefficient 0.69) resembles that of desflurane, ensuring prompt induction of anesthesia and recovery after discontinuation of the anesthetic. Compared with isoflurane, recovery from sevoflurane anesthesia is 3 to 4 minutes faster and the difference is magnified in longer duration surgical procedures (>3 hours) (Fig. 4-4).12 Sevoflurane is nonpungent, has minimal odor, produces bronchodilation similar in degree to isoflurane, and causes the least degree of airway irritation among the currently available volatile anesthetics. For these reasons, sevoflurane, like halothane, is acceptable for inhalation induction of anesthesia.

Sevoflurane may be 100-fold more vulnerable to metabolism than desflurane, with an estimated 3% to 5% of the dose undergoing biodegradation. The resulting metabolites include inorganic fluoride (plasma concentrations exceed those that occur after enflurane) and hexafluoroisopropanol. The chemical structure of sevoflurane is such that it cannot undergo metabolism to an acyl halide. Sevoflurane metabolism does not result in the formation of trifluoroacetylated liver proteins and therefore cannot stimulate the formation of antitrifluoroacetylated protein antibodies. In this regard, sevoflurane differs from halothane, enflurane, isoflurane, and desflurane, all of which are metabolized to reactive acyl halide intermediates with the potential to produce hepatotoxicity as well as cross-sensitivity between drugs.13 Sevoflurane is the least likely volatile anesthetic to form carbon monoxide on exposure to carbon dioxide absorbents. In contrast to other volatile anesthetics, sevoflurane breaks down in the presence of the strong bases present in carbon dioxide absorbents to form compounds that are toxic in animals (Fig. 4-5).5 The principal degradation product is fluoromethyl-2, 2-difluoro-1-(trifluoromethyl) vinyl-ether (compound A). Compound A is a dose-dependent nephrotoxin in rats, causing renal proximal tubular injury. Although this finding is a concern, the levels of these compounds (principally compound A) that occur during administration of sevoflurane to patients are far below speculated toxic levels, even when total gas flows are 1 L per minute.13,14

Xenon
Xenon is an inert gas with many of the characteristics considered important for an ideal inhaled anesthetic.7 MAC is 63% to 71% in humans, suggesting that this gas is more potent than nitrous oxide (MAC 104%).15 MACawake for xenon is 33%.16 Unlike MAC for other volatile anesthetics, there is evidence that xenon MAC is gender-dependent, being less in females.17 Xenon is nonexplosive, nonpungent, odorless, and chemically inert as reflected by absence of metabolism and low toxicity. Unlike other inhaled anesthetics, it is not harmful to the environment because it is prepared by fractional distillation of the atmospheric air. To date, its high cost has hindered its acceptance in anesthesia practice. This disadvantage may be offset to some degree by using low fresh gas flow rates and development of a xenon-recycling system. Nevertheless, even if cost considerations can be negated, acceptance of xenon as a replacement for current inhaled anesthetics (that also share many of the same advantages of xenon) will be based more on evidence that morbidity and mortality is less when this drug is administered during anesthesia.7
Xenon has a blood:gas partition coefficient of 0.115, which is lower than that of other clinically useful anesthetics and even lower than that of nitrous oxide (0.46), sevoflurane (0.69), and desflurane (0.42). Like nitrous oxide, xenon anesthesia results in gas exchange conditions that favor air bubble expansion, which could worsen neurologic injury from venous air embolism.18 Diffusion of xenon into highly compliant bowel occurs but is less compared with nitrous oxide (Fig. 4-6).19 It is possible this minimal effect on bowel may be different when xenon diffuses into less compliant cavities as represented by pneumothorax, pneumoperitoneum, and pneumopericardium. Xenon does not trigger malignant hyperthermia in susceptible swine.20 Emergence from xenon anesthesia, regardless of the duration of anesthesia, is two to three times faster than that from equal-MAC nitrous oxide plus isoflurane or sevoflurane.21 Xenon is a potent hypnotic and analgesic, resulting in suppression of hemodynamic and catecholamine responses to surgical stimulation. Unlike other inhaled and injected anesthetics, xenon does not produce hemodynamic depression in healthy adults. Neuromuscular blocking effects of rocuronium are not different when given during propofol versus xenon anesthesia.22 A risk of recall would seem to be present but has not been observed in small numbers of patients. Like ketamine, xenon exerts antagonist effects at N-methyl-D-aspartate (NMDA) subtypes of glutamate receptors, which have been shown to have both neuroprotective and neurotoxic properties. Xenon is unique among known NMDA antagonists in exhibiting neuroprotection without coexisting psychotomimetic behavioral changes.23 The reason why ketamine and nitrous oxide, but not xenon, produce neurotoxicity may reflect actions on dopaminergic pathways that do not occur in the presence of xenon.

Pharmacokinetics of Inhaled Anesthetics
The pharmacokinetics of inhaled anesthetics describes their (a) absorption (uptake) from alveoli into pulmonary capillary blood, (b) distribution in the body, (c) metabolism, and (d) elimination, principally via the lungs. The pharmacokinetics of volatile anesthetics may be influenced by aging, reflecting decreases in lean body mass and increases in body fat.24 The volume of distribution (Vd) of the central compartment (plasma volume) is smaller, whereas the apparent Vd (steady state) for these drugs in the elderly is larger, especially for those anesthetics most soluble in fat. In addition, impaired pulmonary gas exchange may decrease anesthetic clearance with age. Furthermore, reduced cardiac output in the elderly decreases tissue perfusion, increases time constants, and may be associated with an altered regional distribution of anesthetics. Opposite effects on the pharmacokinetics of inhaled anesthetics might be expected in the very young.
A series of partial pressure gradients beginning at the anesthetic machine serve to propel the inhaled anesthetic across various barriers (alveoli, capillaries, cell membranes) to their sites of action in the CNS. The principal objective of inhalation anesthesia is to achieve a constant and optimal brain partial pressure of the inhaled anesthetic.
The brain and all other tissues equilibrate with the partial pressures of inhaled anesthetics delivered to them by arterial blood (Pa). Likewise, arterial blood equilibrates with the alveolar partial pressures (PA) of anesthetics. This emphasizes that the PA of inhaled anesthetics mirrors the brain partial pressure (PBRAIN) at steady state. This is the reason that PA is used as an index of (a) depth of anesthesia, (b) recovery from anesthesia, and (c) anesthetic equal potency (MAC). It is important to recognize that equilibration between the two phases means the same partial pressure exists in both phases. Equilibration does not mean equality of concentrations in two biophases. Understanding those factors that determine the PA and thus the PBRAIN permits control of the doses of inhaled anesthetics delivered to the brain so as to maintain a constant and optimal depth of anesthesia. This relationship is applicable because volatile anesthetics are only minimally metabolized and as such are excreted from the lung. The availability of an “online” readout of end-tidal partial pressure, which at equilibrium matches brain partial pressure, makes volatile anesthetic dosing easier than intravenous anesthetic dosing.
Determinants of Alveolar Partial Pressure
The PA and ultimately the PBRAIN of inhaled anesthetics are determined by input (delivery) into alveoli minus uptake (loss) of the drug from alveoli into arterial blood (Table 4-2). Input of anesthetics into alveoli depends on the (a) inhaled partial pressure (PI), (b) alveolar ventilation, and (c) characteristics of the anesthetic breathing (delivery) system. Uptake of inhaled anesthetics from alveoli into the pulmonary capillary blood depends on (a) solubility of the anesthetic in body tissues, (b) cardiac output, and (c) alveolar-to-venous partial pressure differences (A-vD).

Inhaled Partial Pressure
A high PI delivered from the anesthetic machine is required during initial administration of the anesthetic. A high initial input offsets the impact of uptake, accelerating induction of anesthesia as reflected by the rate of rise in the PA and thus the PBRAIN. With time, as uptake into the blood decreases, the PI should be decreased to match the decreased anesthetic uptake and therefore maintain a constant and optimal PBRAIN. If the PI is maintained constant with time, the PA and PBRAIN will increase progressively as uptake diminishes.
Concentration Effect
The impact of PI on the rate of rise of the PA of an inhaled anesthetic is known as the concentration effect (Fig. 4-7).25 The concentration effect states that the higher the PI, the more rapidly the PA approaches the PI. The higher PI provides anesthetic molecule input to offset uptake and thus speeds the rate at which the PA increases.

The concentration effect results from (a) a concentrating effect and (b) an augmentation of tracheal inflow.26 The concentrating effect reflects concentration of the inhaled anesthetic in a smaller lung volume due to uptake of all gases in the lung. At the same time, anesthetic input via tracheal inflow is increased to fill the space (void) produced by uptake of gases.
Second-Gas Effect
The second-gas effect reflects the ability of high-volume uptake of one gas (first gas) to accelerate the rate of increase of the PA of a concurrently administered “companion” gas (second gas) (Fig. 4-8).27 For example, the initial large-volume uptake of nitrous oxide accelerates the uptake of companion (second) gases such as oxygen and volatile anesthetics. This increased uptake of the second gas reflects increased tracheal inflow of all the inhaled gases (first and second gases) and higher concentration of the second gas or gases in a smaller lung volume (concentrating effect) due to the high-volume uptake of the first gas (Fig. 4-9).26 Conceptually, the loss of lung volume may be compensated for by decreased expired ventilation as well as increased inspired ventilation (increased tracheal inflow). The implication that extra gas is routinely drawn into the lungs to compensate for loss of lung volume is misleading if compensatory changes include decreased expired ventilation and/or a decrease in lung volume.28


Alveolar Ventilation
Increased alveolar ventilation, like PI, promotes input of anesthetics to offset uptake. The net effect is a more rapid rate of increase in the PA toward the PI and thus induction of anesthesia. In addition to the increased input, the decreased PaCO2 produced by hyperventilation of the lungs decreases cerebral blood flow. Conceivably, the impact of increased input on the rate of rise of the PA would be offset by decreased delivery of anesthetic to the brain. Decreased alveolar ventilation decreases input and thus slows the establishment of a PA and PBRAIN necessary for the induction of anesthesia. The greater the alveolar ventilation to functional residual capacity (FRC) ratio, the more rapid is the rate of increase in the PA. In neonates, this ratio is approximately 5:1 compared with only 1.5:1 in adults, reflecting the greater metabolic rate in neonates compared with adults. As a result, the rate of increase of PA toward the PI and thus the induction of anesthesia is more rapid in neonates than in adults (Fig. 4-10).29

Spontaneous versus Mechanical Ventilation
Inhaled anesthetics influence their own uptake by virtue of dose-dependent depressant effects on alveolar ventilation. This, in effect, is a negative feedback protective mechanism that prevents establishment of an excessive depth of anesthesia (delivery of anesthesia is decreased when ventilation is decreased) when a high PI is administered during spontaneous breathing (Fig. 4-11).30 As anesthetic input decreases in parallel with decreased ventilation, anesthetic present in tissues is redistributed from tissues in which it is present in high concentrations (brain) to other tissues in which it is present in low concentrations (skeletal muscles). When the concentration (partial pressure) in the brain decreases to a certain threshold, ventilation increases and delivery of the anesthetic to the lungs increases. This protective mechanism against development of an excessive depth of anesthesia (anesthetic overdose) is lost when mechanical ventilation of the lungs replaces spontaneous breathing.

Impact of Solubility
The impact of changes in alveolar ventilation on the rate of increase in the PA toward the PI depends on the solubility of the anesthetic in blood. For example, changes in alveolar ventilation influence the rate of increase of the PA of a soluble anesthetic (halothane, isoflurane) more than a poorly soluble anesthetic (nitrous oxide, desflurane, sevoflurane). Indeed, the rate of increase in the PA of nitrous oxide is rapid regardless of the alveolar ventilation. This occurs because uptake of nitrous oxide is limited because of its poor solubility in blood. Conversely, uptake of a more blood-soluble anesthetic is larger, and increasing alveolar ventilation will accelerate the rate at which the PA of the soluble anesthetic approaches the PI. This emphasizes that changing from spontaneous breathing to mechanical (controlled) ventilation of the lungs, which also is likely to be associated with increased alveolar ventilation, will probably increase the depth of anesthesia (PA) produced by a more blood-soluble anesthetic.
Anesthetic Breathing System
Characteristics of the anesthetic breathing system that influence the rate of increase of the PA are the (a) volume of the external breathing system, (b) solubility of the inhaled anesthetics in the rubber or plastic components of the breathing system, and (c) gas inflow from the anesthetic machine. The volume of the anesthetic breathing system acts as a buffer to slow achievement of the PA. High gas inflow rates (5 to 10 L per minute) from the anesthetic machine negate this buffer effect. Solubility of inhaled anesthetics in the components of the anesthetic breathing system initially slows the rate at which the PA increases. At the conclusion of the administration of an anesthetic, however, reversal of the partial pressure gradient in the anesthetic breathing system results in elution of the anesthetic, which slows the rate at which the PA decreases.
Solubility
The solubility of the inhaled anesthetics in blood and tissues is denoted by the partition coefficient (Table 4-3).1,31 A partition coefficient is a distribution ratio describing how the inhaled anesthetic distributes itself between two phases at equilibrium (partial pressures equal in both phases). For example, a blood:gas partition coefficient of 0.5 means that the concentration of inhaled anesthetic in the blood is half that present in the alveolar gases when the partial pressures of the anesthetic in these two phases is identical. Similarly, a brain:blood partition coefficient of 2 indicates a concentration of anesthetic in the brain is twice that in the blood when the partial pressures of anesthetic are identical at both sites.

Partition coefficients may be thought of as reflecting the relative capacity of each phase to accept anesthetic. Partition coefficients are temperature dependent such that the solubility of a gas in a liquid is decreased when the temperature of the liquid increases.
Blood:Gas Partition Coefficients
The rate of increase of the PA toward the PI (maintained constant by mechanical ventilation of the lungs) is inversely related to the solubility of the anesthetic in blood (see Fig. 4-3).32,33 Based on their blood:gas partition coefficients, inhaled anesthetics are categorized traditionally as soluble, intermediately soluble, and poorly soluble (see Table 4-3).1,31 Blood can be considered a pharmacologically inactive reservoir, the size of which is determined by the solubility of the anesthetic in blood. When the blood:gas partition coefficient is high, a large amount of anesthetic must be dissolved in the blood before the Pa equilibrates with the PA. For example, the high blood solubility of methoxyflurane slows the rate at which the PA and Pa increase relative to the PI, and the induction of anesthesia is slow. The impact of high blood solubility on the rate of increase of the Pa can be offset to some extent by increasing the PI above that required for maintenance of anesthesia. This is termed the overpressure technique and may be used to speed the induction of anesthesia, recognizing that sustained delivery of a high PI will result in an anesthetic overdose.
When blood solubility is low, minimal amounts of inhaled anesthetic must be dissolved before equilibration is achieved; therefore, the rate of increase of PA and Pa, and thus onset-of-drug effects such as the induction of anesthesia, are rapid. For example, the inhalation of a constant PI of nitrous oxide, desflurane, or sevoflurane for about 10 minutes results in a PA that is ≥80% of the PI (see Fig. 4-3).32,33 Use of an overpressure technique with sevoflurane is more readily accepted by patients because this anesthetic is less pungent than desflurane. Indeed, one or more vital capacity breaths of high concentrations of sevoflurane (7% with 66% nitrous oxide) may result in loss of the eyelash reflex.34
Associated with the rapid increase in the Pa of nitrous oxide is the absorption of several liters (up to 10 L during the first 10 to 15 minutes) of this gas, reflecting its common administration at inhaled concentrations of 60% to 70%. This high-volume absorption of nitrous oxide is responsible for several unique effects of nitrous oxide when it is administered in the presence of volatile anesthetics or air-containing cavities (see the sections “Concentration Effect,” “Second-Gas Effect,” and “Nitrous Oxide Transfer to Closed Gas Spaces”).
Percutaneous loss of inhaled anesthetics occurs but is too small to influence the rate of increase in the PA.35 With the possible exception of methoxyflurane, the magnitude of metabolism of inhaled anesthetics is too small to influence the rate of increase of the PA. This lack of effect reflects the large excess of anesthetic molecules administered and the saturation, by anesthetic concentrations of inhaled drugs, of enzymes responsible for anesthetic metabolism.36
Blood:gas partition coefficients are altered by individual variations in water, lipid, and protein content and by the hematocrit of whole blood.37,38 For example, blood:gas partition coefficients are about 20% less in blood with a hematocrit of 21% compared with blood with a hematocrit of 43%. Presumably, this decreased solubility reflects the decrease in lipid-dissolving sites normally provided by erythrocytes. Conceivably, decreased solubility of volatile anesthetics in anemic blood would manifest as an increased rate of increase in the PA and a more rapid induction of anesthesia. Ingestion of a fatty meal alters the composition of blood, resulting in an approximately 20% increase in the solubility of volatile anesthetics in blood.39
The solubility of inhaled anesthetics in blood varies with age (Fig. 4-12).40 The blood solubilities of halothane, enflurane, methoxyflurane, and isoflurane are about 18% less in neonates and the elderly compared to young adults. In contrast, the solubility of the less soluble anesthetic sevoflurane (presumably also true for desflurane) is not different in neonates and adults.41

Tissue:Blood Partition Coefficients
Tissue:blood partition coefficients determine uptake of anesthetic into tissues and the time necessary for equilibration of tissues with the Pa. This time for equilibration can be estimated by calculating a time constant (amount of inhaled anesthetic that can be dissolved in the tissue divided by tissue blood flow) for each tissue. One time constant on an exponential curve represents 63% equilibration. Three time constants are equivalent to 95% equilibration. For volatile anesthetics, equilibration between the Pa and PBRAIN depends on the anesthetic’s blood solubility and requires 5 to 15 minutes (three time constants). Fat has an enormous capacity to hold anesthetic, and this characteristic, combined with low blood flow to this tissue, prolongs the time required to narrow anesthetic partial pressure differences between arterial blood and fat. For example, equilibration of fat with isoflurane (three time constants) based on this drug’s fat:blood partition coefficient and an assumed fat blood flow of 2 to 3 mL per minute per 100 g fat is estimated to be 25 to 46 hours. Fasting before elective operations results in transport of fat to the liver, which could increase anesthetic uptake by this organ and modestly slow the rate of increase in the PA of a volatile anesthetic during induction of anesthesia.42
Oil:Gas Partition Coefficients
Oil:gas partition coefficients parallel anesthetic requirements. For example, an estimated MAC can be calculated as 150 divided by the oil:gas partition coefficient. The constant, 150, is the average value of the product of oil:gas solubility and MAC for several inhaled anesthetics with widely divergent lipid solubilities. Using this constant, the calculated MAC for a theoretical anesthetic with an oil:gas partition coefficient of 100 would be 1.5%.
Nitrous Oxide Transfer to Closed Gas Spaces
The blood:gas partition coefficient of nitrous oxide (0.46) is about 34 times greater than that of nitrogen (0.014). This differential solubility means that nitrous oxide can leave the blood to enter an air-filled cavity 34 times more rapidly than nitrogen can leave the cavity to enter blood. As a result of this preferential transfer of nitrous oxide, the volume or pressure of an air-filled cavity increases. Passage of nitrous oxide into an air-filled cavity surrounded by a compliant wall (intestinal gas, pneumothorax, pulmonary blebs, air bubbles) causes the gas space to expand (Fig. 4-13).43 Conversely, passage of nitrous oxide into an air-filled cavity surrounded by a noncompliant wall (middle ear, cerebral ventricles, supratentorial space) causes an increase in intracavitary pressure.

The magnitude of volume or pressure increase is influenced by (a) partial pressure of nitrous oxide, (b) blood flow to the air-filled cavity, and (c) duration of nitrous oxide administration. In an animal model, the inhalation of 75% nitrous oxide doubles the volume of a pneumothorax in 10 minutes (see Fig. 4-7).43 The finding emphasizes the high blood flow to this area. Likewise, air bubbles (emboli) expand rapidly when exposed to nitrous oxide (Fig. 4-14).44 Nevertheless, in neurosurgical patients operated on in the sitting position, 50% nitrous oxide has no measurable effect on the incidence or severity of venous air embolism if its administration is discontinued immediately upon Doppler detection of venous air embolism.45 In contrast to the rapid expansion of a pneumothorax, the increase in bowel gas volume produced by nitrous oxide is slow but can result in an increase in distention and postoperative pain after a 3-hour surgery.

The middle ear is an air-filled cavity that vents passively via the Eustachian tube when pressure reaches 20 to 30 cm H2O. Nitrous oxide diffuses into the middle ear more rapidly than nitrogen leaves, and middle ear pressures may increase if Eustachian tube patency is compromised by inflammation or edema. Indeed, tympanic membrane rupture has been attributed to this mechanism after administration of nitrous oxide. Negative middle ear pressures may develop after discontinuation of nitrous oxide, leading to serous otitis. Nausea and vomiting that may follow general anesthesia may be due to multiple mechanisms, but the role of altered middle ear pressures as a result of nitrous oxide is a consideration.
Intraocular gas bubbles as used for internal retinal tamponade (retinal detachment, macular hole repair, complicated vitrectomy) may persist in the eye for up to 10 weeks following ocular surgery. Administration of nitrous oxide for periods as brief as 1 hour during this time period may result in rapid increases in the volume of intraocular gas within the rigid closed eye that is sufficient to compress the retinal artery with resulting visual loss.46
Cardiopulmonary Bypass
Cardiopulmonary bypass produces changes in blood-gas solubility that depend on the constituents of the priming solution and temperature.47 Nevertheless, the overall effect of hypothermic cardiopulmonary bypass and a crystalloid prime on blood-gas solubility is only 2%. Volatile anesthetics initiated during cardiopulmonary bypass take longer to equilibrate, whereas the same drugs already present when cardiopulmonary bypass is initiated are diluted, potentially decreasing the depth of anesthesia.
Cardiac Output
Cardiac output (pulmonary blood flow) influences uptake and therefore PA by carrying away either more or less anesthetic from the alveoli. An increased cardiac output results in more rapid uptake, so the rate of increase in the PA and thus the induction of anesthesia are slowed. A decreased cardiac output speeds the rate of increase of the PA, because there is less uptake to oppose input.
The effect of cardiac output on the rate of increase in the PA may seem paradoxical. For example, the uptake of more drugs by an increased cardiac output should speed the rate of increase of partial pressures in tissues and thus narrow the A-vD for anesthetics. Indeed, an increase in cardiac output does hasten equilibration of tissue anesthetic partial pressures with the Pa. Nevertheless, the Pa is lower than it would be if cardiac output were normal. Conceptually, a change in cardiac output is analogous to the effect of a change in solubility. For example, doubling cardiac output increases the capacity of blood to hold anesthetic, just as solubility increases the capacity of the same volume of blood.
As with alveolar ventilation, changes in cardiac output most influence the rate of increase of the PA of a soluble anesthetic. Conversely, the rate of increase of the PA of a poorly soluble anesthetic, such as nitrous oxide, is rapid regardless of physiologic deviations of the cardiac output around its normal value. As a result, changes in cardiac output exert little influence on the rate of increase of the PA of nitrous oxide. In contrast, doubling the cardiac output will greatly increase the uptake of soluble anesthetic from alveoli, slowing the rate of increase of the PA. Conversely, a low cardiac output, as with shock, could produce an unexpectedly high PA of a soluble anesthetic.
Volatile anesthetics that depress cardiac output can exert a positive feedback response that contrasts with the negative (protective) feedback response on spontaneous breathing exerted by these drugs. For example, decreases in cardiac output due to an excessive dose of volatile anesthetic results in an increase in the PA, which further increases anesthetic depth and thus cardiac depression. The administration of a volatile anesthetic that depresses cardiac output, plus controlled ventilation of the lungs, results in a situation characterized by unopposed input of anesthetic via alveolar ventilation combined with decreased uptake because of decreased cardiac output. The net effect of this combination of events can be an unexpected, abrupt increase in the PA and an excessive depth of anesthesia.
Distribution of cardiac output will influence the rate of increase of the PA of an anesthetic. For example, increases in cardiac output are not necessarily accompanied by proportional increases in blood flow to all tissues. Preferential perfusion of vessel-rich group tissues when the cardiac output increases results in a more rapid increase in the PA of anesthetic than would occur if the increased cardiac output was distributed equally to all tissues. Indeed, infants have a relatively greater perfusion of vessel-rich group tissues than do adults and, consequently, show a faster rate of increase of the PA toward the PI (see Fig. 4-10).29
Impact of a Shunt
In the absence of an intracardiac or intrapulmonary right-to-left shunt, it is valid to assume that the PA and Pa of inhaled anesthetics are essentially identical. When a right-to-left shunt is present, the diluting effect of the shunted blood on the partial pressure of anesthetic in blood coming from ventilated alveoli results in a decrease in the Pa and a slowing in the induction of anesthesia. Monitoring the end-tidal concentration of anesthetic or carbon dioxide reveals a gradient between the PA and Pa in which the PA underestimates the Pa. A similar mechanism is responsible for the decrease in PaO2 and the gradient between the PA and Pa in the presence of a right-to-left shunt.
The relative impact of a right-to-left shunt on the rate of increase in the Pa depends on the solubility of the anesthetic. For example, a right-to-left shunt slows the rate of increase of the Pa of a poorly soluble anesthetic more than that of a soluble anesthetic.48 This occurs because uptake of a soluble anesthetic offsets dilutional effects of shunted blood on the Pa. Uptake of a poorly soluble drug is minimal, and dilutional effects on the Pa are relatively unopposed. This impact of solubility in the presence of a right-to-left shunt is opposite to that observed with changes in cardiac output and alveolar ventilation. All factors considered, it seems unlikely that a right-to-left shunt alone will alter the speed of induction of anesthesia significantly.
Left-to-right tissue shunts (arteriovenous fistulas, volatile anesthetic–induced increases in cutaneous blood flow) result in delivery to the lungs of blood containing a higher partial pressure of anesthetic than that present in blood that has passed through tissues. As a result, left-to-right shunts offset the dilutional effects of a right-to-left shunt on the Pa. Indeed, the effect of a left-to-right shunt on the rate of increase in the Pa is detectable only if there is a concomitant presence of a right-to-left shunt. Likewise, the effect of a right-to-left shunt on the rate of increase in the PA is maximal in the absence of a left-to-right shunt.
Alveolar-to-Venous Partial Pressure Differences
The A-vD reflects tissue uptake of the inhaled anesthetic. Tissue uptake affects uptake at the lung by controlling the rate of increase of the mixed venous partial pressure of anesthetic. Factors that determine the fraction of anesthetic removed from blood traversing a tissue parallel those factors that determine uptake at the lungs (tissue solubility, tissue blood flow, and arterial-to-tissue partial pressure differences).
Highly perfused tissues (brain, heart, kidneys) in the adult account for <10% of body mass but receive 75% of the cardiac output (Table 4-4). As a result of the small mass and high blood flow, these tissues, known as vessel-rich group tissues, equilibrate rapidly with the Pa. Indeed, after about three time constants, approximately 75% of the returning venous blood is at the same partial pressure as the PA. For this reason, uptake of a volatile anesthetic is decreased greatly after three time constants (5 to 15 minutes, depending on the blood solubility of the inhaled anesthetic), as reflected by a narrowing of the inspired-to-alveolar partial pressure difference. Continued uptake of anesthetic after saturation of vessel-rich group tissues reflects principally the entrance of anesthetic into skeletal muscles and fat. Skeletal muscles and fat represent about 70% of the body mass but receive only about 25% of the cardiac output (see Table 4-4). As a result of the large tissue mass, sustained tissue uptake of the inhaled anesthetic continues and the effluent venous blood is at a lower partial pressure than the PA. For this reason, the A-vD difference for anesthetic is maintained and uptake from the lungs continues, even after several hours of continuous administration of inhaled anesthetics.

The time for equilibration of vessel-rich group tissues is more rapid for neonates and infants than for adults. This difference reflects the greater cardiac output to vessel-rich group tissues in the very young as well as decreased solubility of anesthetics in the tissues of neonates. Furthermore, skeletal muscle bulk comprises a small fraction of body weight in neonates and infants.
Recovery from Anesthesia
Recovery from anesthesia is depicted by the rate of decrease in the PBRAIN as reflected by the PA (Fig. 4-15).32,33 The rate of washout of anesthetic from the brain should be rapid because inhaled anesthetics are not highly soluble in brain and the brain receives a large fraction of the cardiac output. Although similarities exist between the rate of induction and recovery, as reflected by changes in the PA of the inhaled anesthetic, there are important differences between the two events. In contrast to induction of anesthesia, which may be accelerated by the concentration effect, it is not possible to speed the decrease in PA by this mechanism (you cannot administer less than zero). Furthermore, at the conclusion of every anesthetic, the concentration of the inhaled anesthetic in tissues depends highly on the solubility of the inhaled drug and the duration of its administration. This contrasts with tissue concentrations of zero at the initiation of induction of anesthesia. The failure of certain tissues to reach equilibrium with the PA of the inhaled anesthetic during maintenance of anesthesia means that the rate of decrease of the PA during recovery from anesthesia will be more rapid than the rate of increase of the PA during induction of anesthesia (see Figs. 4-3 and 4-15).32,33 Indeed, even after a prolonged anesthetic, skeletal muscles probably, and fat almost certainly, will not have equilibrated with the PA of the inhaled anesthetic. Thus, when the PI of an anesthetic is abruptly decreased to zero at the conclusion of an anesthetic, these tissues initially cannot contribute to the transfer of drug back to blood for delivery to the liver for metabolism or to the lungs for exhalation. As long as gradients exist between the Pa and tissues, the tissues will continue to take up anesthetic. Thus, during recovery from anesthesia, the continued passage of anesthetic from blood to tissues, such as fat, acts to speed the rate of decrease in the PA of that anesthetic. Continued tissue uptake of anesthetic will depend on the solubility of the inhaled anesthetic and the duration of anesthesia, with the impact being most important with soluble anesthetics.49 For example, time to recovery is prolonged in proportion to the duration of anesthesia for soluble anesthetics (halothane and isoflurane), whereas the impact of duration of administration on time to recovery is minimal with poorly soluble anesthetics (sevoflurane and desflurane) (Fig. 4-16).1


Anesthetic that has been absorbed into the components of the anesthetic breathing system will pass from the components back into the gases of the breathing circuit at the conclusion of anesthesia and retard the rate of decrease in the PA of the anesthetic. Likewise, exhaled gases of the patient contain anesthetic that will be rebreathed unless fresh gas flow rates are increased (at least 5 L per minute of oxygen) at the conclusion of anesthesia.
In contrast to the rate of increase of the PA during induction of anesthesia, the rate of decrease in the PA during recovery from anesthesia is not entirely consistent with what might be predicted from the inhaled anesthetic’s blood:gas partition coefficient (Fig. 4-17).50,51 For example, the PA for halothane decreases more rapidly than that for isoflurane and enflurane despite the greater blood solubility of halothane. Similarly, the PA of methoxyflurane decreases below that of enflurane even though methoxyflurane is about six times more soluble in blood than is enflurane. The more rapid decrease in the PA for halothane and methoxyflurane is, in large part, due to the metabolism of these drugs in the liver50,51 (see Chapter 2). This suggests that metabolism can significantly influence the rate of recovery from halothane and methoxyflurane anesthesia. In contrast, the rate of induction of anesthesia is not influenced by the magnitude of metabolism even for drugs such as halothane and methoxyflurane.

Context-Sensitive Half-Time
The pharmacokinetics of the elimination of inhaled anesthetics depends on the length of administration and the blood-gas solubility of the inhaled anesthetic. As with injected anesthetics, it is possible to use computer simulations to determine context-sensitive half-times for volatile anesthetics. In this regard, the time needed for a 50% decrease in anesthetic concentration of enflurane, isoflurane, desflurane, and sevoflurane is <5 minutes and does not increase significantly with increasing duration of anesthesia.52 Presumably, this is a reflection of the initial phase of elimination, which is primarily a function of alveolar ventilation. Determination of other decrement times (80% and 90%) reveals differences between various inhaled anesthetics. For example, the 80% decrement times of desflurane and sevoflurane are <8 minutes and do not increase significantly with the duration of anesthesia, whereas 80% decrement times for enflurane and isoflurane increase significantly after about 60 minutes, reaching plateaus of approximately 30 to 35 minutes. The 90% decrement time of desflurane increases slightly from 5 minutes after 30 minutes of anesthesia to 14 minutes after 6 hours of anesthesia, which is significantly less than sevoflurane (65 minutes), isoflurane (86 minutes), and enflurane (100 minutes) after 6 hours of administration. Based on the simulated context-sensitive half-times and assuming that MAC-awake is 0.5 MAC, there would be little difference in recovery time among these volatile anesthetics when a pure inhalation anesthetic technique is used. The major differences in the rates at which desflurane, sevoflurane, isoflurane, and enflurane are eliminated occur in the final 20% of the elimination process.
Diffusion Hypoxia
Diffusion hypoxia occurs when inhalation of nitrous oxide is discontinued abruptly, leading to a reversal of partial pressure gradients such that nitrous oxide leaves the blood to enter alveoli.53 This initial high-volume outpouring of nitrous oxide from the blood into the alveoli can so dilute the PAO2 that the PaO2 decreases. In addition to dilution of the PAO2 by nitrous oxide, there is also dilution of the PACO2, which decreases the stimulus to breathe.54 This decreased stimulus to breathe exaggerates the impact on PaO2 of the outpouring of nitrous oxide into the alveoli. Outpouring of nitrous oxide into alveoli is greatest during the first 1 to 5 minutes after its discontinuation at the conclusion of anesthesia. Thus, it is common practice to fill the lungs with oxygen at the end of anesthesia to ensure that arterial hypoxemia will not occur as a result of dilution of the PAO2 by nitrous oxide.
Pharmacodynamics of Inhaled Anesthetics
Minimal Alveolar Concentration
MAC of an inhaled anesthetic is defined as that concentration at 1 atmosphere that prevents skeletal muscle movement in response to a supramaximal painful stimulus (surgical skin incision) in 50% of patients.55 MAC is an anesthetic 50% effective dose (ED50). Immobility produced by inhaled anesthetics as measured by MAC is mediated principally by effects of these drugs on the spinal cord and only a minor component of immobility results from cerebral effects.56 For example, in animals, MAC for isoflurane is 1.2% when delivered to the intact animal but delivery of inhaled anesthetic only to the brain results in isoflurane MAC increasing to nearly 3%.57 Further evidence that MAC reflects effects of the inhaled anesthetics at the spinal cord is the observation that decerebration does not change MAC (Fig. 4-18).58

MAC is among the most useful concepts in anesthetic pharmacology as it establishes a common measure of potency (partial pressure at steady state) for inhaled anesthetics. This concept is used to provide uniformity in dosages of inhaled anesthetics, to establish relative amounts of inhaled anesthetics to reach specific endpoints (MACawake), and to guide the search for mechanisms responsible for mechanisms of anesthetic action.59 A unique feature of MAC is its consistency varying only 10% to 15% among individuals. This small degree of pharmacodynamic variability for inhaled anesthetics is unique in pharmacology. The use of equally potent doses (comparable MAC concentrations) of inhaled anesthetics is mandatory for comparing effects of these drugs not only at the spinal cord but also at all other organs (Table 4-5). For example, similar MAC concentrations of inhaled anesthetics produce equivalent depression of the spinal cord, whereas effects on cardiopulmonary parameters may be different for each drug (see Chapter 2). This emphasizes that MAC represents only one point on the dose-response curve of effects produced by inhaled anesthetics and that these dose-response curves are not parallel. It is remarkable though that MACawake, the concentration of anesthetic that prevents consciousness in 50% of persons, is reliably about half of MAC and that MACmemory, the concentration of anesthetic that is associated with amnesia in 50% of patients, is significantly less than MACawake. If this were not the case, an ED50 would not be satisfactory endpoint for clinical anesthesia! A surgeon may tolerate 50% of his or her patients moving but having 50% of patients have awareness under anesthesia would clearly not be acceptable.

Factors that Alter Minimal Alveolar Concentration
Inhalation anesthetic requirements are remarkably uniform in humans, mainly being affected by age and body temperature. MAC allows a quantitative analysis of the effect, if any, of various physiologic and pharmacologic factors on anesthetic requirements (Table 4-6).60,61 For example, increasing age results in a progressive decrease in MAC of about 6% per decade that is similar for all inhaled anesthetics (Figs. 4-19 and 4-20).62,63 MAC is decreased nearly 30% during pregnancy and in the early postpartum period, returning to normal values in 12 to 72 hours.64,65 Nevertheless, gender does not influence MAC (Fig. 4-21).66,67 MAC is increased in women with natural red hair, presumably reflecting mutations of the melanocortin-1 receptor gene and increased pheomelanin concentrations (Fig. 4-22).68 Although most reports describe MAC as independent of the duration of anesthesia, there is evidence that MAC for isoflurane decreases during the administration of anesthesia and the performance of surgery (Fig. 4-23).69 The effect of cardiopulmonary bypass on MAC is uncertain, with some studies showing a decrease, whereas others fail to demonstrate any change.47 Despite prolongation of sleeping times in animals, cyclosporine increases rather than decreases isoflurane MAC.70 MAC is defined by the response to a surgical incision, which is considered to be a supramaximal stimulus. MAC values may vary with the type of stimulus; tetanic stimulation and trapezius squeeze are considered noninvasive stimulation patterns that are relatively equivalent to surgical skin incision, although in contrast to skin incision, these events can be repeated (Fig. 4-24).71 Tracheal intubation requires the highest MAC to prevent skeletal muscle responses and may represent a true supramaximal stimulation (see Fig. 4-24).71







MAC values for inhaled anesthetics are additive.72 For example, 0.5 MAC of nitrous oxide plus 0.5 MAC isoflurane has the same effect at the brain as does a 1 MAC concentration of either anesthetic alone. The strict additivity of the interactions among inhaled anesthetics implies either a common site of action or that anesthetic action occurs with only a small fraction of the binding sites occupied.73
Opioids synergistically decrease anesthetic requirements for volatile anesthetics. For example, 25 minutes after the administration of fentanyl, 3 µg/kg or 6 µg/kg IV, MAC for desflurane is decreased 48% and 68%, respectively.74 Similar decreases in isoflurane MAC are also produced by these doses of fentanyl.75
Dose-response curves for inhaled anesthetics, although not parallel, are all steep. This is emphasized by the fact that a 1 MAC dose prevents skeletal muscle movement in response to a painful stimulus in 50% of patients, whereas a modest increase to about 1.3 MAC prevents movement in at least 95% of patients.
Mechanisms of Anesthetic Action
Meyer-Overton Theory (Critical Volume Hypothesis)
Correlation between the lipid solubility of inhaled anesthetics (oil:gas partition coefficient) and anesthetic potency has historically been presumed to be evidence that inhaled anesthetics act by disrupting the structure or dynamic properties of the lipid portions of nerve membranes. For example, when a sufficient number of molecules dissolve (critical concentration) in crucial hydrophobic sites such as lipid cell membranes, there is distortion of channels necessary for ion flux and the subsequent development of action potentials needed for synaptic transmission. Likewise, changes in the lipid matrix produced by dissolved anesthetic molecules could alter the function of proteins in cell membranes, thus decreasing sodium conductance. Evidence supporting distortion of sodium channels by dissolved anesthetic molecules is the observation that high pressures (40 to 100 atm) partially antagonize the action of inhaled anesthetics (pressure reversal), presumably by returning (compressing) lipid membranes and their sodium channels to their “awake” contour.76
The most compelling evidence against the Meyer-Overton theory of anesthesia is the fact that effects of inhaled anesthetics on the fluidity of lipid bilayers is implausibly small and can generally be mimicked by temperature changes of 1°C.77 Furthermore, not all lipid-soluble drugs are anesthetics, and, in fact, some are convulsants. For example, the observation that, among n-alcohols, dodecanol is anesthetic and decanol is not (for n-alkanes the cutoff is after octane) suggests that anesthetic binding to protein pockets or clefts and not lipid membranes is important in the mechanism of anesthesia. Based on these negative observations, lipid theories have been refined to postulate that specialized domains in membranes (boundary membranes surrounding proteins) are not only particularly sensitive to anesthetics but also are critical to membrane function. Indeed, either binding to proteins or dissolving in lipids can account for the Meyer-Overton correlation.
Stereoselectivity
The effects of inhaled anesthetics on ion channels responsible for neuronal action are readily demonstrated (Fig. 4-25).77 The most definitive evidence that general anesthetics act by binding directly to proteins and not a lipid bilayer comes from observations of stereoselectivity.78 Inhalation anesthetics exist as isomers, and isoflurane has been shown to act stereoselectively on neuronal channels, with the levoisomer being more potent than the dextroisomer in enhancing potassium conductance in neurons79 and in with respect to the loss of righting reflex in animals.80 The relevance to anesthetic mechanisms of the differing effects of enantiomers of volatile anesthetics on in vitro nerve conduction would be supported by parallel changes in MAC in the intact animal. Indeed, in rats, MAC for the levoisomer of isoflurane was 60% more potent than the dextroisomer.81 In contrast, others have not found a significant difference in the effects of the enantiomers of isoflurane and desflurane on anesthetic effects in animals.82 Receptor specificity is also suggested by conversion of an anesthetic to a nonanesthetic by increasing the molecular volume, despite corresponding increases in lipid solubility. Nevertheless, there is evidence that molecular shape (bulkiness) and size provide limited insight into the structure of the anesthetic site of action.83

Potential Mediators of Anesthetic Action
Ionotropic and Metabotropic Receptors
Neurotransmitters signal through two families of receptors designated as ionotropic and metabotropic. Ionotropic receptors are also known as ligand-gated ion channels because the neurotransmitter binds directly to ion channel proteins and this interaction causes opening (gating) of the ion channels allowing transmission of specific ions resulting in changes in membrane potential. Ionotropic receptors are often composed of several subunits. Indeed the γ-aminobuteric acid receptor type A (GABAA) and nicotinic acetylcholine receptors are constructed from large families of evolutionarily related subunits that come together to make pleiomorphic receptors. In contrast, metabotropic receptors are usually monomeric receptors consisting of seven transmembrane segments. Binding of neurotransmitters (acetylcholine) to metabotropic receptors causes activation of guanosine triphosphate binding proteins (G proteins) associated with the receptors, and these G proteins act as second messengers to activate other signaling molecules such as protein kinases, or potassium or calcium channels.56
Inhaled anesthetics do not seem to stimulate the release of endogenous opioids and do not suppress ventilatory responses to surgical stimulation at concentrations that suppress movement. The fact that small doses of opioids decrease MAC reflects their ability to provide an effect (analgesia) that is not present with inhaled anesthetics alone.
Inhibitory Ligand-Gated and Voltage-Gated Channels (Glycine and GABAA Receptors)
Glycine receptors are major mediators of inhibitory neurotransmission in the spinal cord and may mediate part of the immobility produced by inhaled anesthetics.84 Their spinal localization and potentiation by volatile anesthetics at clinical concentrations is consistent with their being a target for mediating immobility as defined by MAC. Intravenous and intrathecal administration of strychnine, a glycine receptor antagonist increases MAC. An argument in favor of a role for glycine receptor potentiation in mediating MAC is the fact that strychnine only reverses the effect of ketamine (which does not affect glycinergic currents) to a threshold while it affects dose dependent reversal of volatile anesthetic effect.
Although β3-subunit containing GABAA receptors mediate hypnosis and part of the immobility produced by injected anesthetics (propofol, etomidate),85 there is evidence that GABAA receptors do not mediate immobility produced by inhaled anesthetics. In this regard, although GABAA receptors are potentiated at MAC concentrations of all clinically used volatile anesthetics, their enhancement of GABAA receptor activation minimally influences MAC.86
Glutamate (NMDA, AMPA, and Kainate Receptors)
Inhaled anesthetics decrease excitatory neurotransmission in the CNS. Glutamate is the principal excitatory neurotransmitter in the mammalian CNS. Glutamate receptors include G protein–coupled receptors and the ligand-gated receptors (NMDA, AMPA, and kainate). NMDA receptors may mediate some important behavioral effects of inhaled anesthetics. Volatile anesthetics separate into two different classes in terms of their efficacy for NMDA blockade. At 1 MAC concentration, different volatile anesthetics inhibit NMDA receptors by between 12% and 74% with those anesthetics having cation-π interactions having the greatest NMDA blockade.87
Two-Pore Potassium Channels
Two-pore potassium channels are intrinsic membrane receptor/ion channels that normally act to maintain the cell’s resting potential and responds to internal stimuli such as a change in pH. Several members of this family have been recently found to be sensitive to volatile anesthetics at clinically used concentrations. TREK and TASK channel activity is potentiated by volatile anesthetics in an agent-specific manner.88 TASK-3 receptors are an anesthetic sensitive receptor that play a role in maintaining theta oscillations in the EEG that are associated with anesthesia and natural deep sleep.89
Voltage-Gated Sodium Channels
Despite the historical notion that inhaled anesthetics do not block axonal conduction and thus voltage-dependent sodium channels, it is now clear that there are many subtypes of sodium channels. Although sodium channels that mediate axonal conduction are not significantly effected at MAC concentrations of volatile anesthetics, those that modulate the release of neurotransmitter may be more sensitive to anesthetics. There is evidence that by interacting with specific subtypes of sodium channel that are expressed at the presynaptic junction, these drugs can inhibit the release of neurotransmitters, particularly glutamate.90 Indeed, intravenous administration of lidocaine, which is a nonspecific sodium channel blocker, decreases MAC.
Hyperpolarization-Activated Cyclic Nucleotide–Gated Channels
Hyperpolarization-activated cyclic nucleotide–gated (HCN) channels are voltage-gated ion channels that are expressed throughout the body but are particularly important in regulating rhythmogenicity in heart and brain. There is some suggestion that halothane may affect HCN channels in motor neurons to induce anesthetic immobility.91
Mechanism of Immobility
MAC is based on the characteristic ability of inhaled drugs to produce immobility by virtue of actions of these drugs principally on the spinal cord rather than on higher centers.56 The observation that immobility during noxious stimulation does not correlate with electroencephalographic activity reflects the fact that cortical electrical activity does not control motor responses to noxious stimulation. Effects of inhaled anesthetics on the spinal cord leading to immobility are diverse. In this regard, inhaled anesthetics depress excitatory AMPA and NMDA receptor-mediated currents by actions independent of inhibitory GABAA and glycine receptor-mediated currents. Actions on two-pore potassium channels may also be important in producing immobility. Conversely, cholinergic receptors do not seem to exert a significant role in anesthetic-induced immobility at the spinal cord level.92 Likewise, although opioids and stimulation of α2 adrenergic receptors (clonidine) decrease MAC, it is unlikely that immobility produced by inhaled anesthetics is due to activation of these receptors.66 Inhaled anesthetics do not act via opioid receptors. Overall, no inhaled anesthetic action on a single group of receptors yet described can explain immobility, and immobility as a result of concurrent actions on many receptors is unlikely.56,93
Mechanism of Anesthesia-Induced Unconsciousness
A comprehensive explanation of the mechanism by which volatile anesthetics cause loss of consciousness (suppression of awareness) is not known.94 Hypnosis is typically studied in animal models as loss of righting reflex. In human studies, it can be measured as loss of response to command and in the presence of neuromuscular blockers the spared arm technique uses a tourniquet to block muscle relaxant access to an arm, which is then used to indicate consciousness. Subtle differences in the clinical effects of inhaled anesthetics may be attributed to distinct actions on a number of critical molecular targets. There is evidence that loss of consciousness (hypnosis), amnesia, and the response to skin incision (immobility as defined by MAC) are not a single continuum of increasing anesthetic depth but rather separate phenomena.95,96 Combining these two observations, it has been proposed that general anesthesia is a process requiring a state of unconsciousness of the brain (produced by volatile or injected anesthetics) plus immobility in response to a noxious stimulus (surgical skin incision) that is mediated by the action of volatile anesthetics on the spinal cord administered at concentrations equivalent to MAC for that drug.
It has been proposed that clinical anesthesia is a hierarchical process in which afferent sensory impulses are diminished by some drugs (opioids, regional anesthesia, ketamine) while the central activating systems are depressed by another mechanism and sometimes other drugs (benzodiazepines, barbiturates, propofol, etomidate, ketamine and volatile anesthetics) and motor reflexes are depressed by yet another mechanism (volatile anesthetics, propofol, etomidate, barbiturates). As defined by MAC, only agents which depress motor reflexes in addition to the other actions can be properly considered general anesthetics but many agents are able to provide one or the other behavioral outcome and can be used as part of a general anesthetic regimen. Volatile anesthetics have been called total anesthetics because they can be used as a single agent to provide general anesthesia. It was long assumed that they decreased pain transmission, but after more thorough consideration, it appears that volatile anesthetics have a biphasic dose response for nociceptive influences such that they are increased at very low volatile anesthetic concentrations (about 10% MAC) and they diminish thereafter.97
Presynaptic inhibition of neurotransmitter release may explain how certain inhaled anesthetics can inhibit synaptic transmission. Inhibition of neurotransmitter release by inhaled anesthetics appears to be mediated by inhibition of neurosecretion rather than by inhibition of transmitter synthesis or storage. The mechanism by which anesthetics act to inhibit neurotransmitter release might reflect actions at ion channels that regulate the probability of neurotransmitter release or could act on the machinery of release itself. As mentioned earlier, halogenated volatile anesthetics inhibit some types of sodium channels in native neurons and in heterologous expression systems.98 Although sodium channels can presynaptically modulate the release of both glutamate and GABA, their effect on the release of glutamate is greater than on the release of GABA.99
Comparative Pharmacology of Gaseous Anesthetic Drugs
Inhaled anesthetics evoke different pharmacologic effects at comparable percentages of MAC concentrations, emphasizing that dose-response curves for these drugs are not necessarily parallel. Measurements obtained from normothermic volunteers exposed to equal potent concentrations of inhaled anesthetics during controlled ventilation of the lungs to maintain normocapnia have provided the basis of comparison for pharmacologic effects of these drugs on various organ systems.100 In this regard, it is important to recognize that surgically stimulated patients who have other confounding variables may respond differently than healthy volunteers (Table 4-7).

Desflurane and sevoflurane provide one specific advantage over other currently available potent inhaled anesthetics.4 Their lower blood and tissue solubility permit more precise control over the induction of anesthesia and a more rapid recovery when the drug is discontinued. Most of the other properties of these new volatile anesthetics resemble their predecessors, especially at concentrations of ≤1 MAC.
Central Nervous System Effects
Mental impairment is not detectable in volunteers breathing 1,600 ppm (0.16%) nitrous oxide or 16 ppm (0.0016%) halothane.101 It is therefore unlikely that impairment of mental function in the personnel who work in the operating room using modern anesthetic scavenging techniques can result from inhaling trace concentrations of anesthetics. Reaction times do not increase significantly until 10% to 20% nitrous oxide is inhaled.102
Cerebral metabolic oxygen requirements are decreased in parallel with drug-induced decreases in cerebral activity. Drug-induced increases in cerebral blood flow may increase intracranial pressure (ICP) in patients with space-occupying lesions. The effects of desflurane and sevoflurane on the CNS do not differentiate these inhaled anesthetics from the older inhaled drugs.
Electroencephalogram
Volatile anesthetics in concentrations of <0.4 MAC similarly increase the frequency and voltage on the electroencephalogram (EEG). This enhancement is representative of the “excitement stage” of anesthesia. At about 0.4 MAC, there is an abrupt shift of high-voltage activity from posterior to anterior portions of the brain.103 Cerebral metabolic oxygen requirements also begin to decrease abruptly at about 0.4 MAC. It is likely that these changes reflect a transition from wakefulness to unconsciousness. Furthermore, amnesia probably occurs at this dose of volatile anesthetic. As the dose of volatile anesthetic approaches 1 MAC, the frequency on the EEG decreases and maximum voltage occurs. During administration of isoflurane, burst suppression appears on the EEG at about 1.5 MAC, and at 2 MAC, electrical silence predominates.104 Electrical silence does not occur with enflurane, and only unacceptably high concentrations of halothane (>3.5 MAC) produce this effect. The effects of nitrous oxide on the EEG are similar to those produced by volatile anesthetics. Slower frequency and higher voltage develop on the EEG as the dose of nitrous oxide is increased or when nitrous oxide is added to a volatile anesthetic to provide a greater total MAC concentration.
Desflurane and sevoflurane cause dose-related changes in the EEG similar to those that occur with isoflurane.4 With desflurane, the EEG progresses from an initial increase in frequency and lowering of voltage at low anesthetic concentrations to increased voltage at anesthetizing concentrations. Higher concentrations of desflurane produce decreasing voltage and increasing periods of electrical silence with an isoelectric EEG at 1.5 to 2.0 MAC. The addition of nitrous oxide to a given level of anesthesia with desflurane causes little or no change in the EEG.
Seizure Activity
Enflurane can produce fast frequency and high voltage on the EEG that often progresses to spike wave activity that is indistinguishable from changes that accompany a seizure. This EEG activity may be accompanied by tonic-clonic twitching of skeletal muscles in the face and extremities. The likelihood of enflurane-induced seizure activity is increased when the concentration of enflurane is >2 MAC or when hyperventilation of the lungs decreases the PaCO2 to <30 mm Hg. Repetitive auditory stimuli can also initiate seizure activity during the administration of enflurane. There is no evidence of anaerobic metabolism in the brain during seizure activity produced by enflurane. Furthermore, in an animal model, enflurane does not enhance preexisting seizure foci, with the possible exception being certain types of myoclonic epilepsy and photosensitive epilepsy.105
Isoflurane does not evoke seizure activity on the EEG, even in the presence of deep levels of anesthesia, hypocapnia, or repetitive auditory stimulation. Indeed, isoflurane possesses anticonvulsant properties; it is able to suppress seizure activity produced by flurothyl.106 An undocumented speculation is that the greater MAC value for enflurane compared with its isomer, isoflurane, reflects the need for a higher concentration to suppress the stimulating effects of enflurane in the CNS.
Desflurane and sevoflurane, like isoflurane, do not produce evidence of convulsive activity on the EEG either at deep levels of anesthesia or in the presence of hypocapnia or auditory stimulation. Nevertheless, there are reports of pediatric patients with epilepsy and otherwise healthy adults who developed EEG evidence of seizure activity during sevoflurane anesthesia.107,108 Sevoflurane can suppress convulsive activity induced with lidocaine.
The administration of nitrous oxide may increase motor activity with clonus and opisthotonus even in clinically used concentrations.109 When nitrous oxide is administered in high concentrations in a hyperbaric chamber, abdominal muscle rigidity, catatonic movements of extremities, and periods of skeletal muscle activity may alternate with periods of skeletal muscle relaxation, clonus, and opisthotonus.110 Although very rare, tonic-clonic seizure activity has been described after administration of nitrous oxide to an otherwise healthy child.111 Animals suspended by their tails may experience seizures in the first 15 to 90 minutes after discontinuation of nitrous oxide but not of volatile anesthetics.112 It is possible that these withdrawal seizures reflect acute nitrous oxide dependence. In patients, delirium or excitement during recovery from anesthesia that included nitrous oxide could reflect this phenomenon.
Evoked Potentials
Volatile anesthetics cause dose-related decreases in the amplitude and increases in the latency of the cortical component of median nerve somatosensory evoked potentials, visual evoked potentials, and auditory evoked potentials.113,114 Decreases in amplitude are more marked than increases in latencies. In the presence of 60% nitrous oxide, waveforms adequate for monitoring cortical somatosensory evoked potentials are present during administration of 0.50 to 0.75 MAC halothane and 0.5 to 1.0 MAC enflurane and isoflurane.115 Peri-MAC concentrations of desflurane (0.5 to 1.5 MAC) increasingly depress somatosensory evoked potentials in patients.4 Even nitrous oxide alone may decrease the amplitude of cortical somatosensory evoked potentials.
Mental Function and Awareness
By definition, inhaled anesthetics cause loss of response to verbal command at MAC-awake concentrations. Subtle effects on mental function (learning) may occur at lower anesthetic concentrations (0.2 MAC).116 Gaseous anesthetics may not be equally effective in preventing awareness. For example, 0.4 MAC isoflurane prevents recall and responses to commands, whereas nitrous oxide requires greater than 0.5 to 0.6 MAC to produce similar effects. Surgical stimulation may increase the anesthetic requirement to prevent awareness.
Cerebral Blood Flow
Volatile anesthetics produce dose-dependent increases in cerebral blood flow (CBF). The magnitude of this increase is dependent on the balance between the drug’s intrinsic vasodilatory actions and vasoconstriction secondary to flow-metabolism coupling. Volatile anesthetics administered during normocapnia in concentrations of >0.6 MAC produce cerebral vasodilation, decreased cerebral vascular resistance, and resulting dose-dependent increases in CBF (Fig. 4-26).100 This drug-induced increase in CBF occurs despite concomitant decreases in cerebral metabolic requirements. Sevoflurane has an intrinsic dose-dependent cerebral vasodilatory effect but this effect is less than that of isoflurane.117 Desflurane and isoflurane are similar in terms of increases in CBF and the preservation of reactivity to carbon dioxide (Fig. 4-27).118 Nitrous oxide also increases CBF, but its restriction to concentrations of <1 MAC limits the magnitude of this change. In fact, nitrous oxide may be a more potent cerebral vasodilator than an equipotent dose of isoflurane alone in humans.119


Anesthetic-induced increases in CBF occur within minutes of initiating administration of the inhaled drug and whether blood pressure is unchanged or decreased, emphasizing the cerebral vasodilating effects of these drugs. Animals exposed to halothane demonstrate a time-dependent return to baseline from the previously increased CBF beginning after about 30 minutes and reaching predrug levels after about 150 minutes.120 This normalization of CBF reflects a concomitant increase in cerebral vascular resistance that is not altered by α- or β-adrenergic blockade and is not the result of changes in the pH of the cerebrospinal fluid.121
Unlike the decay in CBF with time observed in animals, CBF remains increased relative to cerebral metabolic oxygen requirements for as long as 4 hours during administration of halothane, isoflurane, or sevoflurane to patients during surgery (see Fig. 4-26).122 Furthermore, in these patients, isoflurane possesses greater capability to maintain global CBF relative to cerebral metabolic oxygen requirements than does halothane or sevoflurane (Fig. 4-28).122 An unchanging EEG during this period suggests that CBF is increased over time without decay rather than a parallel change in CBF and cerebral metabolic oxygen requirements.

In animals, autoregulation of CBF in response to changes in systemic blood pressure is retained during administration of 1 MAC isoflurane but not halothane (Fig. 4-29).100,123 Indeed, increases in systemic blood pressure produce smaller increases in brain protrusion during administration of isoflurane and enflurane compared with halothane.123 It is speculated that loss of autoregulation during administration of halothane is responsible for the greater brain swelling seen in animals anesthetized with this drug. Inhaled anesthetics including desflurane and sevoflurane do not alter autoregulation of CBF as reflected by the responsiveness of the cerebral circulation to changes in PaCO2.118,124,125 For example, cerebrovascular carbon dioxide reactivity is described as intact during administration of 1 MAC desflurane.126 Nevertheless, others describe impairment of autoregulation by desflurane with 1.5 MAC nearly abolishing autoregulation.127

Cerebral Metabolic Oxygen Requirements
Inhaled anesthetics produce dose-dependent decreases in cerebral metabolic oxygen requirements that are greater during the administration of isoflurane than with an equivalent MAC concentration of halothane.128 When the EEG becomes isoelectric, an additional increase in the concentration of the volatile anesthetics does not produce further decreases in cerebral metabolic oxygen requirements. The greater decrease in cerebral metabolic oxygen requirements produced by isoflurane may explain why CBF is not predictably increased by this anesthetic at concentrations lower than 1 MAC. For example, decreased cerebral metabolism means less carbon dioxide is produced, which thus opposes any increase in CBF. It is conceivable that isoflurane could evoke unexpected increases in CBF if administered to a patient in whom cerebral metabolic oxygen requirements were already decreased by drugs. Desflurane and sevoflurane decrease cerebral metabolic oxygen requirements similar to isoflurane.
Cerebral Protection
In animals experiencing temporary focal ischemia, there is no difference in neurologic outcome when cerebral function is suppressed by isoflurane or thiopental if systemic blood pressure is maintained.129 In humans undergoing carotid endarterectomy, the CBF at which ischemic changes appear on the EEG is lower during administration of isoflurane than during enflurane or halothane (Fig. 4-30).130 Although neurologic outcome is not different based on the volatile anesthetic administered, these data suggest that relative to enflurane and halothane, isoflurane may offer a degree of cerebral protection (blunts necrotic processes resulting from cerebral ischemia) from transient incomplete regional cerebral ischemia during carotid endarterectomy.131 Unchanged CBF and decreased cerebral metabolic oxygen requirements during isoflurane-induced controlled hypotension for clipping of cerebral aneurysms indicates that global cerebral oxygen supply-demand balance is favorably altered in patients anesthetized with this anesthetic.132

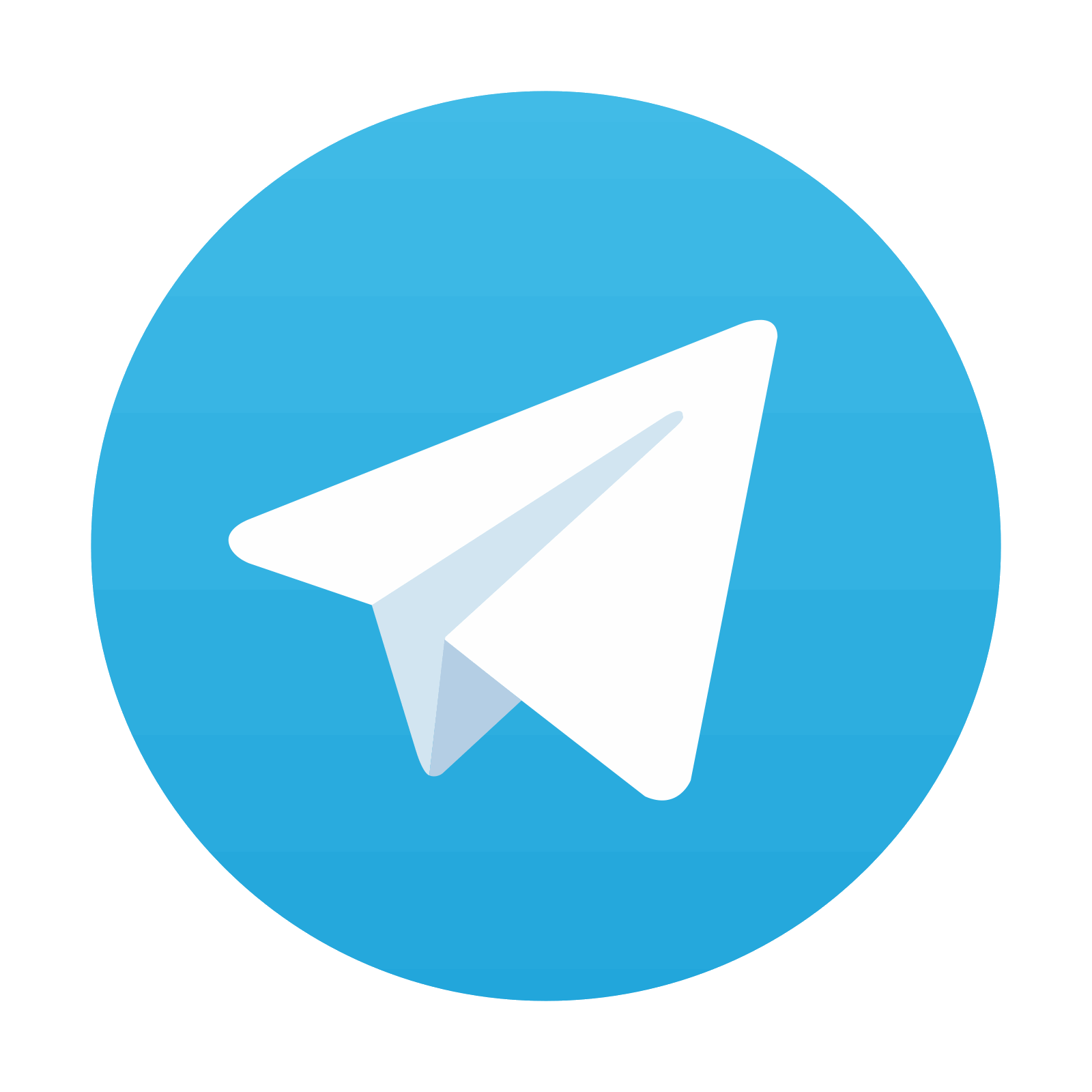
Stay updated, free articles. Join our Telegram channel
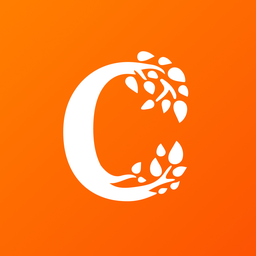
Full access? Get Clinical Tree
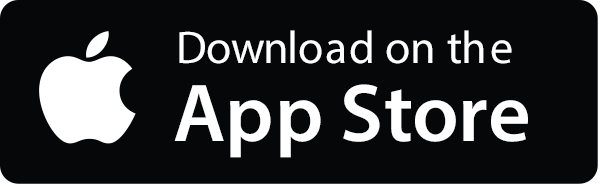
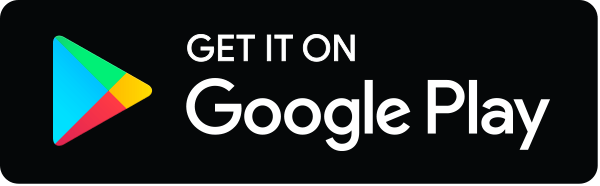