Inhaled Anesthetics
Thomas J. Ebert
Larry LindenbauM
Key Points














Related Matter
Inhaled Anesthetic Formulas
Inhaled Anesthetic Rate of Rise

Sevoflurane, desflurane, and isoflurane are the most popular potent inhaled anesthetics used in adult surgical procedures (Fig. 17-1). In pediatric cases, sevoflurane is the most commonly employed. Although there are many similarities in terms of the overall effects of the volatile anesthetics (e.g., they all have a dose-dependent effect to decrease blood pressure [BP]), there are some unique differences that might influence the clinician’s selection process depending on the patient’s health and the surgical procedure. Discussion of these three most popular inhaled anesthetics provides the major emphasis of this chapter. For the sake of completeness and for historical perspective related to metabolism and toxicity, comments on halothane, enflurane, and methoxyflurane are also included.
Pharmacokinetic Principles
Kety in 1950 was the first to examine the pharmacokinetics of inhaled agents in a systematic fashion.1 Eger accomplished much of the early research in the field, leading to his landmark text on the subject in 1974.2 The inhaled anesthetics differ substantially from nearly all other drugs because they are gases given via inhalation. This makes their pharmacokinetics unique as well, and discussion of pharmacokinetic principles of currently used agents necessary for understanding and predicting their effects.
Drug pharmacology is classically divided into two disciplines, pharmacodynamics and pharmacokinetics. Pharmacodynamics can be defined as what drugs do to the body. It describes the desired and undesired effects of drugs, as well as the cellular and molecular changes leading to these effects. Pharmacokinetics can be defined as what the body does to drugs. It describes where drugs go, how they are transformed, and the cellular and molecular mechanisms underlying these processes.
Tissues are often grouped into hypothetical compartments based on perfusion. An important implication of different compartments and perfusion rates is the concept of redistribution. After a given amount of drug is administered, it reaches highly perfused tissue compartments first, where it can equilibrate rapidly and exert its effects. With time, however, compartments with lower perfusion rates receive the drug and additional equilibria are established between blood and these tissues. As the tissues with lower perfusion rates absorb the drug, maintenance of equilibria throughout the body requires drug transfer from highly perfused compartments back into the bloodstream. This lowering of drug concentration in one compartment by delivery into another compartment is called redistribution.
In discussions of the inhaled anesthetics, the absorption phase is usually called uptake, the metabolic phase is usually called biotransformation, and the excretion phase is usually called elimination.
Unique Features of Inhaled Anesthetics
Speed, Gas State, and Route of Administration
The inhaled anesthetics are among the most rapidly acting drugs in existence, and when administering a general anesthetic, this speed provides a margin of safety. The ability to quickly increase or decrease anesthetic levels as necessary can mean the difference
between an anesthetic state and an anesthetic misadventure. Speed also means efficiency. Rapid induction and recovery may lead to faster operating room turnover times, shorter recovery room stays, and earlier discharges to home.
between an anesthetic state and an anesthetic misadventure. Speed also means efficiency. Rapid induction and recovery may lead to faster operating room turnover times, shorter recovery room stays, and earlier discharges to home.
Technically, of the inhaled anesthetics only N2O and xenon are true gases, while the so-called potent agents are the vapors of volatile liquids. But for simplicity, all of them are referred to as gases because they are all in the gas phase when administered via the lungs. As gases, none deviate significantly from ideal gas behavior. These agents are all nonionized and have low molecular weights. This allows them to diffuse rapidly without the need for facilitated diffusion or active transport from bloodstream to tissues. The other advantage of gases is that they can be delivered to the bloodstream via a unique route available in all patients: the lungs.
Speed, gaseous state, and route of administration combine to form the major beneficial feature of the inhaled anesthetics: The ability to decrease plasma concentrations as easily and as rapidly as they are increased.
Physical Characteristics of Inhaled Anesthetics


where P is partial pressure. Equilibration is a result of three factors:
Inhaled anesthetics are gases rapidly transferred bidirectionally via the lungs to and from the bloodstream and subsequently to and from CNS tissues as partial pressures equilibrate.
Table 17-1. Physiochemical Properties of Volatile Anesthetics
Property
Sevoflurane
Desflurane
Isoflurane
Enflurane
Halothane
N2O
Boiling point (°C)
59
24
49
57
50
−88
Vapor pressure at 20°C (mm Hg)
157
669
238
172
243
38,770
Molecular weight (g)
200
168
184
184
197
44
Oil:gas partition coefficient
47
19
91
97
224
1.4
Blood:gas partition coefficient
0.65
0.42
1.46
1.9
2.50
0.46
Brain:blood solubility
1.7
1.3
1.6
1.4
1.9
1.1
Fat:blood solubility
47.5
27.2
44.9
36
51.1
2.3
Muscle:blood solubility
3.1
2.0
2.9
1.7
3.4
1.2
MAC in O2 30–60 years, at 37°C PB 760 (%)
1.8
6.6
1.17
1.63
0.75
104
MAC in 60–70% N2O (%)
0.66
2.38
0.56
0.57
0.29
MAC, >65 years (%)
1.45
5.17
1.0
1.55
0.64
—
Preservative
No
No
No
No
Thymol
No
Stable in moist CO2 absorber
No
Yes
Yes
Yes
No
Yes
Flammability (%) (in 70% N2O/30% O2)
10
17
7
5.8
4.8
Recovered as metabolites (%)
2–5
0.02
0.2
2.4
20
MAC, minimum alveolar concentration; N2O, nitrous oxide.
Plasma and tissues have a low capacity to absorb the inhaled anesthetics relative to the amount we can deliver to the lungs, allowing us to quickly establish or abolish anesthetizing concentrations of anesthetic in the bloodstream and ultimately the CNS.
Metabolism, excretion, and redistribution of the inhaled anesthetics are minimal relative to the rate at which they are delivered or removed from the lungs. This permits easy maintenance of blood and CNS concentrations.
The so-called permanent gases, such as oxygen and nitrogen, exist only as gases at ambient temperatures. Gases such as N2O can be compressed into liquids under high pressure at ambient temperature. Most potent volatile anesthetics are liquids at ambient temperature and pressure. If the system in which the volatile liquid resides is a closed container, molecules of the substance will equilibrate between the liquid and gas phases. At equilibrium, the pressure exerted by molecular collisions of the gas against the container walls is the vapor pressure. One important property of vapor pressure is that as long as any liquid remains in the container, the vapor pressure is independent of the volume of that liquid. As with any gas, however, vapor pressure is proportional to temperature.
For all of the potent agents, at 20°C the vapor pressure is below atmospheric pressure. If the temperature is raised, the vapor pressure increases. The boiling point of a liquid is the temperature at which its vapor pressure exceeds atmospheric pressure in an open container. Desflurane is bottled in a special container because its boiling point of 23.5°C makes it boil at typical room temperatures. Boiling does not occur within the bottle because it is countered by buildup of vapor pressure within the bottle, but once opened to air, the desflurane would quickly boil away. The bottle is designed to allow transfer of desflurane from bottle to vaporizer without exposure to the atmosphere.
Gases in Mixtures
For any mixture of gases in a closed container, each gas exerts a pressure proportional to its fractional mass. This is its partial pressure.
The sum of the partial pressures of each gas in a mixture of gases equals the total pressure of the entire mixture (Dalton’s law).
The sum of the partial pressures of each gas in a mixture of gases equals the total pressure of the entire mixture (Dalton’s law).

Another way to state this is that each gas in a mixture of gases at a given volume and temperature has a partial pressure that is the pressure it would have if it alone occupied the volume. The entire mixture behaves just as if it were a single gas according to the ideal gas law.
Gases in Solution
Partial pressure of a gas in solution is a bit complex because pressure can only be measured in the gas phase, while in solution the amount of gas is measured as a concentration. Partial pressure of a gas in solution refers to the pressure of the gas in the gas phase (if it were present) in equilibrium with the liquid. It is important to talk of partial pressures, however, because gases equilibrate based on partial pressures, not concentrations.
Gas molecules within a liquid interact with solvent molecules to a much larger extent than do molecules in the gas phase. Solubility is the term used to describe the tendency of a gas to equilibrate with a solution, hence determining its concentration in solution. Henry’s law expresses the relationship of concentration of a gas in solution to the partial pressure of the gas with which the solution is in equilibrium:

where Cg is concentration of gas in solution, k is a solubility constant, and Pg is the partial pressure of the gas. From Eq. 17-3 one can see that doubling the pressure of a gas doubles its concentration in solution. A more clinically useful expression of solubility is the solubility coefficient, λ:

where V = volume. This equation states that for any gas in equilibrium with a liquid, a certain volume of that gas dissolves in a given volume of liquid.
The principles of partial pressures and solubility apply in mixtures of gases in solution. That is, the concentration of any one gas in a mixture of gases in solution depends on two factors: (1) its partial pressure in the gas phase in equilibrium with the solution, and (2) its solubility within that solution.
The implications of these properties are that anesthetic gases administered via the lungs diffuse into blood until the partial pressures in alveoli and blood are equal. The concentration of anesthetic in the blood depends on the partial pressure at equilibrium and the blood solubility. Likewise, transfer of anesthetic from blood to target tissues also proceeds toward equalizing partial pressures, but at this interface there is no gas phase. A partial pressure still exists to force anesthetic molecules out of solution and into a gas phase but there is no gas phase because blood (outside the lungs) and tissues are like closed, liquid-filled containers. Remember the principle: The partial pressure of a gas in solution represents the pressure that the gas in equilibrium with the liquid would have if a gas phase existed in contact with the liquid phase.
The concentration of anesthetic in target tissue depends on the partial pressure at equilibrium and the target tissue solubility. Because inhaled anesthetics are gases, and because partial pressures of gases equilibrate throughout a system, monitoring the alveolar concentration of inhaled anesthetics provides an index of their effects in the brain.
In summary:
Inhaled anesthetics equilibrate based on their partial pressures in each tissue (or tissue compartment), not based on their concentrations.
The partial pressure of a gas in solution is defined by the partial pressure in the gas phase with which it is in equilibrium. Where there is no gas phase the partial pressure reflects a force to escape out of solution.
The concentration of anesthetic in a tissue depends on its partial pressure and tissue solubility.
Finally, the particular terminology used when referring to gases in the gas phase or absorbed in plasma or tissues is important. Inspired concentrations or fractional volumes of inhaled anesthetic are typically used rather than partial pressure. Partial pressure is expressed in millimeters of mercury (mm Hg) or torr (1 torr = 1 mm Hg) or kilopascals (kPa). For most drugs, concentration is expressed as mass (milligram [mg]) per volume (milliliter [mL]), but it can also be expressed in percent by weight or volume. Since volume of a gas in the gas phase is directly proportional to mass according to the ideal gas law, it is easier to express this fractional concentration as a percent by volume. In the gas phase, fractional concentration is equal to the partial pressure divided by ambient pressure, usually atmospheric, or:

Anesthetic Transfer: Machine to Central Nervous System
When the fresh gas flow and the vaporizer are turned on, fresh gas with a fixed fractional concentration of anesthetic leaves the fresh gas outlet and mixes with the gas in the circuit—the bag, tubing, absorbent canister, and piping. It is immediately diluted to a lower fractional concentration, then slowly rises as this compartment equilibrates with the fresh gas flow. With spontaneous patient ventilation by mask, the anesthetic gas passes from circuit to airways. The fractional concentration of anesthetic leaving the circuit is designated as FI (fraction inspired). In the lungs the gas comprising the dead space in the airways (trachea, bronchi) and the alveoli further dilutes the circuit gas. The fractional concentration of anesthetic present in the alveoli is FA (fraction alveolar). The anesthetic then passes across the alveolar–capillary membrane and dissolves in pulmonary blood according to the partial pressure of the gas and its blood solubility. It is further diluted and travels via bulk blood flow throughout the vascular tree. The anesthetic then passes via simple diffusion from blood to tissues as well as between tissues.
The vascular system delivers blood to three physiologic tissue groups: the vessel-rich group (VRG), the muscle group, and the fat group. The VRG includes the brain, heart, kidney, liver, digestive tract, and glandular tissues. The percent of body mass and perfusion of each group are shown in Table 17-2. The CNS tissues of the VRG are referred to as tissues of desired effect. The other tissues of the VRG that comprise the compartment are referred to as tissues of undesired effects. The tissues of the muscle and fat groups comprise the tissues of accumulation.
Anesthetic is delivered most rapidly to the VRG because of high blood flow. Here it diffuses according to partial pressure gradients. CNS tissue takes in the anesthetic according to the tissue solubility, and at a high enough tissue concentration,
unconsciousness and anesthesia are achieved. Increasing CNS tissue concentrations cause progressively deeper stages of anesthesia. As this is occurring, anesthetic is also distributing to other VRG tissues. Also coincident with delivery to the CNS, anesthetic is being delivered—albeit more slowly because of lower perfusion—to muscle and fat, where it accumulates and may affect the speed of emergence from the anesthetic. In reality, the fat solubilities provide little influence on emergence in cases lasting <4 hours since the delivery of anesthetic to fat tissue is extremely slow as a result of low blood flow. The concentration of inhaled anesthetic in a given tissue at a particular time during the administration depends not only on tissue blood flow, but also on tissue solubility, which governs how the inhaled anesthetics partition themselves between blood and tissue. Partitioning depends on the relative solubilities of the anesthetic for each compartment. These relative solubilities are expressed by a partition coefficient, δ, which is the ratio of dissolved gas (by volume) in two-tissue compartments at equilibrium. Some of the partition coefficients for the inhaled anesthetics are shown in Table 17-1.
unconsciousness and anesthesia are achieved. Increasing CNS tissue concentrations cause progressively deeper stages of anesthesia. As this is occurring, anesthetic is also distributing to other VRG tissues. Also coincident with delivery to the CNS, anesthetic is being delivered—albeit more slowly because of lower perfusion—to muscle and fat, where it accumulates and may affect the speed of emergence from the anesthetic. In reality, the fat solubilities provide little influence on emergence in cases lasting <4 hours since the delivery of anesthetic to fat tissue is extremely slow as a result of low blood flow. The concentration of inhaled anesthetic in a given tissue at a particular time during the administration depends not only on tissue blood flow, but also on tissue solubility, which governs how the inhaled anesthetics partition themselves between blood and tissue. Partitioning depends on the relative solubilities of the anesthetic for each compartment. These relative solubilities are expressed by a partition coefficient, δ, which is the ratio of dissolved gas (by volume) in two-tissue compartments at equilibrium. Some of the partition coefficients for the inhaled anesthetics are shown in Table 17-1.
Table 17-2. Distribution of Cardiac Output by Tissue Group | ||||||||||||||||
---|---|---|---|---|---|---|---|---|---|---|---|---|---|---|---|---|
|
Uptake and Distribution
FA/FI



FFGO is the fraction of inspired anesthetic in the gas leaving the fresh gas outlet (i.e., the vaporizer setting), T is time, and τ is a time constant. The time constant is simply the volume or “capacity” of the circuit (VC) divided by the fresh gas flow (FGF) or τ = VC/FGF. For example, if the bag, tubing, absorbent canister, and piping comprise 8 L, and the fresh gas flow is 2 L, the time constant τ = 8/2 = 4. One of the characteristics of first-order kinetics is that 95% of maximum is reached after three time constants—in this case, 3 × 4 = 12 minutes.
Since 12 minutes is relatively long, starting with a higher FFGO can increase the rate of rise of FI. Using the earlier example with τ = 4, by first-order kinetics 63% of maximum is reached after one time constant, or 4 minutes. To attain an FI of 2% at 4 instead of 12 minutes, the FFGO can be set to 3.2% (2% divided by 0.63) and then lowered to 2% at the 4-minute mark.
Other ways to speed the increase in FI include increasing the fresh gas flow, thus decreasing τ. Furthermore, the rebreathing bag can be collapsed prior to starting the fresh gas flow, such that the capacity in the circuit (VC) is less, which also decreases τ. Finally, at high flows (>4 L/min) there is far less mixing because fresh gas pushes “old” gas out of the circuit via the pop-off valve before complete mixing occurs, causing FI to increase at a greater rate; this is the most important factor in rapidly increasing FI to the desired concentration.
One factor that delays the rate of rise of FI is that CO2 absorbent can adsorb and decompose the inhaled anesthetics. From a practical standpoint, this does not affect the rate of rise in FI to a significant extent compared with other factors. Another factor that delays the rate of rise of FI is solubility of the inhaled anesthetics in some of the plastic and rubber parts of the anesthesia circuit. This absorption has been quantified, but plays only a small role in decreasing the rate of rise of FI.
Rise in FA in the Absence of Uptake
The rate of rise in FI discussed earlier assumes that no anesthetic is mixing with gas in the patient’s lungs. In reality, circuit gas mixes with exhaled gases from the lung with each breath, thus lowering FI within the circuit. If high fresh gas flows (>4 L/min), which produce a high volume of gas at the desired concentration, are used, little mixing with exhaled air occurs and FI is relatively fixed. In this situation, circuit gas enters the lungs where it mixes
with alveolar gas. If there were no blood flow to the lungs, FA would rise in a fashion analogous to FI; that is:
with alveolar gas. If there were no blood flow to the lungs, FA would rise in a fashion analogous to FI; that is:

In this equation, τ is the time constant for alveolar rise in anesthetic concentration and equals the functional residual capacity (FRC) of the patient’s lungs divided by minute ventilation, [V with dot above]A. There are two ways to speed the equilibration of FA with FI, that is, to decrease τ. One way is to increase minute ventilation, and the other is to decrease FRC. Both of these methods can be used to speed induction by mask; the patient can exhale deeply before applying the mask (to decrease the initial FRC), and the patient can breathe deeply and rapidly (to increase) after the mask is applied. Importantly, high alveolar ventilation relative to uptake from the lungs to the bloodstream generates the initial high slope to the curves shown in Figure 17-2.
One of the reasons that pediatric inductions by spontaneous breathing of inhaled anesthetics are so much quicker than adult inductions is that the low FRC relative to [V with dot above]A of children makes for a low time constant, and hence a more rapid increase in FA/FI. One important caveat about the relationship of FA to FRC is that FRC includes airway dead space; thus, in reality, FA by Eq. 17-7 is not just the concentration of inhaled anesthetic in the alveoli but also the concentration in the entire lung. However, it is simply called the alveolar concentration because the dead space in the airways is relatively insignificant and only the alveolar gas is exchanging anesthetic with the blood.
Rise in FA in the Presence of Uptake
Anesthetics are soluble in tissues, thus uptake of anesthetic from alveoli to blood is again characterized by first-order kinetics:

where

Here, PB is the barometric pressure and the time constant, τ, equals “capacity” (volume of anesthetic dissolved in blood at the desired alveolar partial pressure) divided by flow (volume of anesthetic delivered per unit time). For any given flow of anesthetic into the system, this capacity for the more soluble halothane is greater than the capacity for the less soluble desflurane; thus, τ for halothane is greater than that for desflurane. The more soluble the inhaled anesthetic, the larger the capacity of the blood and tissues for that anesthetic, and the longer it takes to saturate at any given delivery rate.
Table 17-3. Factors that Increase or Decrease the Rate of Rise of FA/FI | |||||||||||||||||||||
---|---|---|---|---|---|---|---|---|---|---|---|---|---|---|---|---|---|---|---|---|---|
|
The most important factor in the rate of rise of FA/FI is uptake of anesthetic from the alveoli into the bloodstream. The rate of rise of FA/FI (especially the position of the “knees” in the curves of Fig. 17-2) reflects the speed at which alveolar anesthetic (FA) equilibrates with that being delivered to the lungs (FI). Since there is uptake from alveoli to blood, FA is not solely a function of FI and time. The greater the uptake, the slower the rate of rise of FA/FI, and vice versa. Since uptake is proportional to tissue solubility, the less soluble the anesthetic (such as desflurane), the lesser its uptake and the faster it reaches equilibrium, PA = Pblood = PCNS.
Consider a hypothetical example. Suppose that halothane and desflurane are soluble in blood, but insoluble in all other tissues. Suppose further that total lung capacity and blood volume were both 5 L. If a fixed volume of anesthetic is delivered to the lungs (by asking the patient to take one deep breath and hold it), according to the blood:gas partition coefficients for halothane (2.5) and desflurane (0.42), 71.4% of the delivered halothane will be transferred to the blood while 28.6% remains in the alveoli (71.4/28.6 = 2.5). In contrast, 29.6% of the desflurane will be transferred to the blood while 70.4% remains in the alveoli (29.6/70.4 = 0.42). Therefore, 2.4 times (71.4/29.6) more halothane than desflurane (by volume or number of molecules) will be transferred from alveoli to bloodstream before partial pressures equilibrate. At equilibrium, the alveolar partial pressures of halothane and desflurane are 28.6% and 70.4% of their inhaled values, respectively. This means that FA rises faster with desflurane than halothane, as does FA/FI.
Blood uptake of anesthetic is expressed by the equation:

where [V with dot above]B is blood uptake, δb/g is the blood:gas partition coefficient, Q is cardiac output, PA is alveolar partial pressure of anesthetic, Pv is mixed venous partial pressure of anesthetic, and PB is barometric pressure. This is the Fick equation applied to blood uptake of inhaled anesthetics. The greater the value of [V with dot above]B, the greater the uptake from alveoli to blood, and the slower the rise in FA/FI.
From the preceding paragraphs, the parameters that increase or decrease the rate of rise in FA/FI during induction can now be clearly delineated and these important factors have been substantiated in experimental models (Table 17-3).
Distribution (Tissue Uptake)
The maximum FA/FI at a given inspired concentration of anesthetic, cardiac output, and minute ventilation depends entirely on the solubility of that drug in the blood as characterized by the blood:gas partition coefficient δb/g. This can be seen in the time curves for the rise in FA/FI during induction for the various inhalation anesthetics shown in Figure 17-2. The first “knee” in each curve in Figure 17-2 represents the point at which the rapid rise in Pv begins to taper off; that is, when significant inhaled anesthetic concentrations begin to build up in the bloodstream because of distribution to and equilibration with the various tissue compartments.
As blood is equilibrating with alveolar gas, it also begins to equilibrate with the VRG, muscle, and, more gradually, the fat compartments based on perfusion. Muscle is not that different from the VRG, having partition coefficients that range from 1.2 (N2O) to 3.4 (halothane), just under a threefold difference; and for each anesthetic except N2O, the muscle partition coefficient is approximately double that for the VRG. Although both VRG and muscle are lean tissues, the muscle compartment equilibrates far more slowly than the VRG. The explanation comes in part due to the mass of the compartments relative to perfusion. The perfusion of the VRG is about 75 mL/min/100 g of tissue, whereas it is only 3 mL/min/100 g of tissue in the muscle (Table 17-2). This 25-fold difference in perfusion between VRG (especially brain) and muscle means that even if the partition coefficients were equal, the muscle would still take 25 times longer to equilibrate with blood.
Fat is perfused to a lesser extent than muscle and its time for equilibration with blood is considerably slower because the partition coefficients are so much greater. All of the potent agents are highly lipid-soluble. Partition coefficients range from 27 (desflurane) to 51 (halothane). On average, the solubility for these agents is about 25 times greater in fat than in the VRG group. Thus, fat equilibrates far more slowly with the blood and does not play a significant role in determining speed of induction. After long anesthetic exposures (>4 hours), the high saturation of fat tissue may play a role in delaying emergence.
Nitrous oxide represents an exception. Its partition coefficients are fairly similar in each tissue: It does not accumulate to any great extent and is not a very potent anesthetic. Its utility lies as an adjunct to the potent agents, and as a vehicle to speed induction.
Metabolism
Data suggest that enzymes responsible for biotransformation of inhaled anesthetics become saturated at less than anesthetizing doses of these drugs, such that metabolism plays little role in opposing induction. It may, however, have some significance to recovery from anesthesia, as discussed later.
Overpressurization and the Concentration Effect
There are several ways to speed uptake and induction of anesthesia with the inhaled anesthetics. The first is overpressurization, which is analogous to an intravenous bolus. This is the administration of a higher partial pressure of anesthetic than the alveolar concentration (FA) actually desired for the patient. Inspired anesthetic concentration (FI) can influence both FA and the rate of rise of FA/FI. The greater the inspired concentration of an inhaled anesthetic, the greater the rate of rise. This concentration effect has two components: The concentrating effect and an augmented gas inflow effect.
For example, consider the administration of 10% anesthetic (10 parts anesthetic and 90 parts other gas) to a patient in whom 50% of the anesthetic in the alveoli is absorbed by the blood. In this case, five parts (0.5 × 10) anesthetic remain in the alveoli, five parts enter the blood, and 90 parts remain as other alveolar gas. The alveolar concentration is now 5/(90 + 5) = 5.3%. Consider next administering 50% anesthetic with the same 50% uptake. Now 25 parts anesthetic remain in alveoli, 25 parts pass into blood, and 50 parts remain as other alveolar gas. The alveolar concentration becomes 25/(50 + 25) = 33%. Giving five times as much anesthetic will lead to a 33%/5.3% = 6.2 times greater alveolar concentration. The higher the FI, the greater the effect. Thus N2O, typically given in concentrations of 50% to 70%, has the greatest concentrating effect. This is why the FA/FI versus time curve in Figure 17-2 rises the most quickly with N2O, even though desflurane has a slightly lower blood:gas solubility.
This is not the complete picture; there is yet another factor to consider. As gas is leaving the alveoli for the blood, new gas at the original FI is entering the lungs to replace that which is taken up by the blood. This other aspect of the concentration effect has been called augmented gas inflow. Again, take the example of 10% anesthetic delivered with 50% uptake into the bloodstream. The five parts anesthetic absorbed by the bloodstream are replaced by gas in the circuit that is still 10% anesthetic. The five parts anesthetic and 90 parts other gas left in the lungs mix with five parts replacement gas, or 5 × 0.10 = 0.5 parts anesthetic. Now the alveolar concentration is (5 + 0.5)/(100) = 5.5% (as compared to 5.3% without augmented inflow). For 50% anesthetic and 50% uptake, 25 parts of anesthetic removed from the alveoli are replaced with 25 parts of 50% anesthetic, giving a new alveolar concentration of (25 + 12.5)/(100) = 37.5% (as compared to 33% without augmented inflow). Thus, 5 times the FI leads to 37.5/5.5 = 6.8 times greater FA (compared to 6.2 times without augmented gas inflow). Of course, this cycle of absorbed gas being replaced by fresh gas inflow is continuous and has a finite rate, so our example is a simplification.
Second Gas Effect
A special case of concentration effect applies to administration of a potent anesthetic with N2O, that is, two gases simultaneously. Along with the concentration of potent agent in the alveoli via its uptake, there is further concentration via the uptake of N2O, a process called the second gas effect. The principle is simple (Figs. 17-3 and 17-4). Consider, for example, administering 2% of a potent anesthetic in 70% N2O and 28% oxygen. In this case, N2O, with its extremely high partial pressure (despite low solubility), partitions into the blood more rapidly than the potent anesthetic, decreasing the alveolar N2O concentration by some amount (e.g., by 50%). Ignoring uptake of the potent anesthetic, the uptake of N2O is 35 parts, leaving 35 parts N2O, 28 parts O2, and two parts potent agent in the alveoli. The anesthetic gas is now present in the alveoli at a concentration of 2/(2 + 35 + 28) = 3.1%. The potent agent has been concentrated and FA is increased.
Ventilation Effects
As indicated by Figure 17-2 and Table 17-3, inhaled anesthetics with very low tissue solubility have an extremely rapid rise in FA/FI with induction. This suggests that there is very little room to
improve this rate by increasing or decreasing ventilation, which is consistent with the experimental evidence shown in Figure 17-5. The greater the solubility of an inhaled anesthetic, the more rapidly it is absorbed by the bloodstream, such that anesthetic delivery to the lungs may be rate limiting to the rise in FA/FI. Therefore, for more soluble anesthetics, augmentation of anesthetic delivery by increasing minute ventilation also increases the rate of rise in FA/FI.
improve this rate by increasing or decreasing ventilation, which is consistent with the experimental evidence shown in Figure 17-5. The greater the solubility of an inhaled anesthetic, the more rapidly it is absorbed by the bloodstream, such that anesthetic delivery to the lungs may be rate limiting to the rise in FA/FI. Therefore, for more soluble anesthetics, augmentation of anesthetic delivery by increasing minute ventilation also increases the rate of rise in FA/FI.
Spontaneous minute ventilation is not static, however, and to the extent that the inhaled anesthetics depress spontaneous ventilation with increasing inspired concentration, [V with dot above]A will decrease and so will the rate of rise of FA/FI. This is demonstrated in Figure 17-5. This negative feedback should not be considered a
drawback of the inhaled anesthetics because the respiratory depression produced at high anesthetic concentrations essentially slows the rise in FA/FI. This might arguably add a margin of safety in preventing an overdose. Controlled ventilation does not offer this margin of safety.
drawback of the inhaled anesthetics because the respiratory depression produced at high anesthetic concentrations essentially slows the rise in FA/FI. This might arguably add a margin of safety in preventing an overdose. Controlled ventilation does not offer this margin of safety.
Perfusion Effects
As with ventilation, cardiac output is not static during the course of induction. For the less soluble agents, changes in cardiac output do not affect the rate of rise of FA/FI to a great extent, but for the more soluble agents the effect is noticeable, as seen in Figure 17-6. However, as inspired concentration increases, greater cardiovascular depression reduces anesthetic uptake and actually increases the rate of rise of FA/FI. This positive feedback can rapidly lead to profound cardiovascular depression. Figure 17-6 presents experimental data in which lower cardiac outputs lead to a much more rapid rise in FA/FI when [V with dot above]A is held constant. This more rapid rise is greater than can be accounted for by concentration effect alone.
Ventilation–Perfusion Mismatching
Ventilation and perfusion are normally fairly well matched in healthy patients, such that PA (alveolar partial pressure)/PI and Pa (arterial partial pressure)/PI are the same curve. However, if significant intrapulmonary shunt occurs, as in the case of inadvertent bronchial intubation, the rate of rise of alveolar and arterial anesthetic partial pressures can be affected. The effects, however, depend on the solubility of the anesthetic, as seen in Figure 17-7. Ventilation of the intubated lung is dramatically increased while perfusion increases slightly. The non-intubated lung receives no ventilation, while perfusion decreases slightly. For the less-soluble anesthetics, increased ventilation of the intubated lung cannot appreciably increase alveolar partial pressure relative to inspired concentration on that side, but alveolar partial pressure on the non-intubated side is essentially zero. Pulmonary mixed venous blood, therefore, comprises nearly equal parts of blood containing normal amounts of anesthetic and blood containing no anesthetic; that is, diluted relative to normal. Thus the rate of rise in Pa relative to PI is significantly reduced. There is less total anesthetic uptake, so the rate of rise of PA relative to PI increases even though induction of anesthesia is slowed because CNS partial pressure equilibrates with Pa. For the more soluble anesthetics, increased ventilation of the intubated lung does increase the alveolar partial pressure relative to inspired concentration on that side. Pulmonary venous blood from the intubated side contains a higher concentration of anesthetic that lessens the dilution by blood from the non-intubated side. Thus, the rate of rise of Pa/PI is not as depressed as that for the less soluble anesthetics, and induction of anesthesia is less delayed relative to normal.
Elimination
Percutaneous and Visceral Loss
Although the loss of inhaled anesthetics via the skin is very small, it does occur and the loss is the greatest for N2O. These anesthetics also pass across gastrointestinal viscera and the pleura. During open abdominal or thoracic surgery there is some anesthetic loss via these routes. Relative to losses by all other routes, losses via percutaneous and visceral routes are insignificant.
Diffusion Between Tissues
Using more elaborate mathematical modeling of inhaled anesthetic pharmacokinetics than presented here, several laboratories have derived a five-compartment model that best describes tissue compartments. These compartments are the alveoli, the VRG, the muscle, the fat, and one additional compartment. Current opinion is that this fifth compartment represents adipose tissue adjacent to lean tissue that receives anesthetic via intertissue diffusion. This transfer of anesthetic is not insignificant, and may account for up to one-third of uptake during long administration.
Exhalation and Recovery
Recovery from anesthesia, like induction, depends on anesthetic solubility, cardiac output, and minute ventilation. Solubility is the primary determinant of the rate of fall of FA (Fig. 17-8). The greater the solubility of inhaled anesthetic, the larger the capacity for absorption in the bloodstream and tissues. The “reservoir” of anesthetic in the body at the end of administration depends on tissue solubility (which determines the capacity) and the dose and duration of anesthetic (which determine how much of that capacity is filled). Recovery from anesthesia, or “washout,” is usually expressed as the ratio of expired fractional concentration of anesthetic (FA) to the expired concentration at time zero (FA0) when the anesthetic was discontinued (or FA/FA0). Elimination curves of low- and high-soluble anesthetics are shown in Figure 17-9. The longer the duration of a highly soluble anesthetic, the greater the reservoir of anesthetic in the body, and the higher the curve seen in the right half of Figure 17-9. This effect is nearly absent with low-soluble agents such as N2O, desflurane, and sevoflurane.3
There are two major pharmacokinetic differences between recovery and induction. First, whereas overpressurization can increase the speed of induction, there is no “underpressurization.” Both induction and recovery rates depend on the PA to Pv gradient, and PA can never fall below zero. Second, whereas all tissues begin induction with zero anesthetic, each begins recovery with quite different anesthetic concentrations. The VRG tissues begin recovery with the same anesthetic partial pressure as that in alveoli, since PCNS = Pblood = Palveoli. The partial pressures in muscle and fat depend on the inspired concentration during anesthesia, the duration of administration, and the anesthetic tissue solubilities. As long as an arterial-to-tissue partial pressure gradient exists, these tissues will absorb anesthetic—especially fat, since it is a huge potential reservoir whose anesthetic partial pressures are typically low after hours of anesthesia. After discontinuation of anesthesia, muscle and fat may continue to absorb anesthetic, even hours later. The redistribution continues until blood/alveolar anesthetic partial pressure falls below tissue partial pressure. This redistribution causes the early rate of decline in alveolar anesthetic concentration during recovery to exceed its early rate of increase during induction.
Because VRG tissues are highly perfused and washout of anesthetic is mostly via elimination from these tissues early in recovery, all anesthetics, regardless of duration of administration, have approximately the same rate of elimination to 50% of FA0. Unfortunately, halving the CNS concentration of anesthetic is rarely sufficient for waking the patient. More commonly, 80% to 90% of inhaled anesthetic must be eliminated before emergence. At these
amounts of washout, the more soluble anesthetics are eliminated more slowly than less soluble agents.
amounts of washout, the more soluble anesthetics are eliminated more slowly than less soluble agents.
Diffusion Hypoxia
During recovery from anesthesia, washout of high concentrations of N2O can lower alveolar concentrations of oxygen and carbon dioxide, a phenomenon called diffusion hypoxia. The resulting alveolar hypoxia can cause hypoxemia, and alveolar hypocarbia can depress respiratory drive, which may exacerbate hypoxemia. It is therefore appropriate to initiate recovery from N2O anesthesia with 100% oxygen rather than less concentrated O2/air mixtures.
Clinical Overview of Current Inhaled Anesthetics
Isoflurane

Desflurane
Desflurane is a fluorinated methyl ethyl ether that differs from isoflurane by just one atom: A fluorine atom is substituted for a chlorine atom on the α-ethyl component of isoflurane (Fig. 17-1). The process of complete fluorination of the ether molecule has several effects. It decreases blood and tissue solubility (the blood:gas solubility of desflurane equals that of N2O), which results in a loss of potency (the MAC of desflurane is five times higher than isoflurane). It also results in a high vapor pressure owing to decreased intermolecular attraction, requiring the development of a new heated, pressurized vaporizer requiring electrical power to deliver a regulated concentration of desflurane as a gas. One of the advantages of desflurane is the near-absent metabolism to serum trifluoroacetate. This makes immune-mediated hepatitis a rare occurrence. Desflurane is the most pungent of the volatile anesthetics and, if administered via the face mask, results in coughing, salivation, breath holding, and laryngospasm. In extremely dry CO2 absorbers, desflurane (and to a lesser extent isoflurane, enflurane, and sevoflurane) degrades to form carbon monoxide. Desflurane has the lowest blood:gas solubility of the potent volatile anesthetics; moreover, its fat solubility is roughly half that of the other volatile anesthetics. Thus, desflurane requires less downward titration in long surgical procedures to achieve a rapid emergence by virtue of decreased tissue saturation. This may be particularly advantageous in the morbidly obese patient.4 Desflurane has been associated with tachycardia, hypertension, and, in select cases, myocardial ischemia when used in high concentrations or when rapidly increasing the inspired concentration (in the presence of minimal opioid adjuvants).
Sevoflurane
Sevoflurane is a sweet-smelling, completely fluorinated methyl isopropyl ether (Fig. 17-1). Its vapor pressure is roughly one-fourth that of desflurane and it can be used in a conventional vaporizer. The blood:gas solubility of sevoflurane is second only to desflurane in terms of potent volatile anesthetics. Sevoflurane is approximately half as potent as isoflurane, and some of the preservation of potency, despite fluorination, is because of the bulky propyl side chain on the ether molecule. Its minimal odor, lack of pungency, and potent bronchodilating characteristics make sevoflurane administration via the face mask for induction of anesthesia in both children and adults a reasonable alternative to IV anesthetics. Sevoflurane is half as potent a coronary vasodilator as isoflurane, but is 10 to 20 times more vulnerable to metabolism than isoflurane. The metabolism of sevoflurane results in inorganic fluoride; the increase in plasma fluoride after sevoflurane administration has not been associated with renal-concentrating defects. Unlike other potent volatile anesthetics, sevoflurane is not metabolized to trifluoroacetate; rather, it is metabolized to an acyl halide (hexafluoroisopropanol). This does not stimulate formation of antibodies associated with hepatitis.
Sevoflurane can form carbon monoxide during exposure to dry CO2 absorbents, and can cause a canister fire via an exothermic reaction in dry absorbent. New generic versions of sevoflurane have the potential to break down to hydrogen fluoride when exposed to metal compounds because of their lack of adequate water in the formulation. Sevoflurane also breaks down in the presence of the carbon dioxide absorber to form a vinyl halide called compound A. Compound A has been shown to be a dose-dependent nephrotoxin in rats, but has not been associated with renal injury in human volunteers or patients, with or without renal impairment, even when fresh gas flows are 1 L/min or less.
Xenon
Xenon is an inert gas occurring naturally in air at 0.05 parts per million (ppm). Xenon has received considerable interest in the last few years because it has many characteristics approaching those of an “ideal” inhaled anesthetic.5,6 It has a quick onset and offset, minimal effects on the cardiovascular and neural systems, and it is not a trigger for malignant hyperthermia (MH). It is not a pollutant or an occupational hazard, and does not add to global warming or the greenhouse gas effect. Its blood:gas partition coefficient is 0.115,7 and unlike the other potent volatile anesthetics (except methoxyflurane), xenon provides some degree of analgesia. This action is likely due to N-Methyl-D-aspartate (NMDA) receptor inhibition. The MAC of xenon in humans is 71%, which might prove to be a limitation, although this is less than the MAC for N2O. It is nonexplosive, nonpungent, and odorless, and thus can be inhaled with ease. In addition, it does not produce significant myocardial depression or alter coronary blood flow.5,8 Because of its scarcity and high cost, new anesthetic systems are being developed to provide for recycling of xenon. If this proves to be too difficult from either a technical or patient safety standpoint, it may be necessary to use it in a very low, or closed, fresh gas flow system to reduce wastage.
Nitrous Oxide

combination with opioids or volatile anesthetics during the conduct of general anesthesia. At room temperature it is a gas; its boiling point is −88.48°C (Table 17-1). It is stored in cylinders and condensed to 50 atmospheres, leading to a pressure of 745 psi. This pressure is maintained in the cylinders until no liquid remains. Only cylinder weight is a reliable indicator of the volume of N2O in storage tanks. Although not flammable, N2O will support combustion. Unlike the potent volatile anesthetics in clinical use, N2O does not produce significant skeletal muscle relaxation, but it does have analgesic effects. Despite a long track record of use, controversy has surrounded N2O in four areas: Its role in postoperative nausea and vomiting; its potential toxic effects on cell function via inactivation of vitamin B12; its adverse effects related to absorption and expansion into air-filled structures and bubbles; and lastly, its effect on embryonic development. The one concern that seems most valid and most clinically relevant is the ability of N2O to expand air-filled spaces because of its greater solubility in blood compared to nitrogen. This might explain the increased post-operative nausea and vomiting (PONV) associated with N2O use since closed gas spaces reside in the middle ear and bowel. Other closed spaces may occur as a result of disease or surgery, such as a pneumothorax. Since nitrogen in air-filled spaces cannot be removed readily via the bloodstream, N2O delivered to a patient diffuses from the blood into these closed gas spaces quite easily until the partial pressure equals that of the blood and alveoli. Compliant spaces will continue to expand until sufficient pressure is generated to oppose further N2O flow into the space. The higher the inspired concentration of N2O, the higher the partial pressure required for equilibration.
Seventy-five percent N2O can expand a pneumothorax to double or triple its size in 10 and 30 minutes, respectively. Air-filled cuffs of pulmonary artery catheters and endotracheal tubes also expand with the use of N2O, possibly causing tissue damage via increased pressure in the pulmonary artery or trachea, respectively.9,10 Accumulation of N2O in the middle ear can diminish hearing postoperatively11 and is contraindicated for tympanoplasty because the increased pressure can dislodge a tympanic graft.
Neuropharmacology of Inhaled Anesthetics
Minimum Alveolar Concentration

The 95% confidence ranges for MAC are approximately ±25% of the listed MAC values. Manufacturer’s recommendations and clinical experience establish 1.2 to 1.3 times MAC as a dose that consistently prevents patient movement during surgical stimuli. Loss of consciousness typically precedes the absence of stimulus-induced movement by a wide margin. Although 1.2 to 1.3 MAC values do not absolutely ensure the defining criteria for brain anesthesia (the absence of self-awareness and recall), vast clinical experience suggests it is extremely unlikely for a patient to have awareness or recall of the surgical incision at these anesthetic concentrations, barring other conditions that increase MAC in that patient (Table 17-4).
Table 17-4. Factors that Increase Minimum Alveolar Concentration | |
---|---|
|
Concentrations of inhaled anesthetics that provide loss of self-awareness and recall are about 0.4 to 0.5 MAC. Several lines of reasoning support the assertion. First, most patients receiving only 50% N2O (approximately 0.4 to 0.5 MAC), as in a typical dentist’s office, will have no recall of their procedure during N2O administration. Second, various studies have shown that a shift in electroencephalogram (EEG) dominance to the anterior leads, that is, the shift from self-aware to non self-aware, accompanies loss of consciousness, and in primates, the EEG shift and loss of consciousness occur at 0.5 MAC.12 Third, in dogs, loss of consciousness accompanies a sudden nonlinear fall in cerebral metabolic rate (CMRO2) at approximately 0.5 MAC (Fig. 17-10).
MAC values can be established for any measurable response. For example, MAC-awake and MAC-BAR. MAC-awake is the alveolar concentration of anesthetic at which a patient opens his
or her eyes to command, and it varies from 0.15 to 0.5 MAC.13 Interestingly, transition from awake to unconscious and back typically shows some hysteresis in that it quite consistently takes 0.4 to 0.5 MAC to lose consciousness, but less than that (as low as 0.15 MAC) to regain it. This may be because of the speed of alveolar wash-in versus wash-out.14 MAC-BAR is the alveolar concentration of anesthetic that blunts adrenergic responses to noxious stimuli. It has been approximated at 50% higher than standard MAC. 15 MAC values also have been established for discreet levels of EEG activity, such as onset of burst suppression or isoelectricity.
or her eyes to command, and it varies from 0.15 to 0.5 MAC.13 Interestingly, transition from awake to unconscious and back typically shows some hysteresis in that it quite consistently takes 0.4 to 0.5 MAC to lose consciousness, but less than that (as low as 0.15 MAC) to regain it. This may be because of the speed of alveolar wash-in versus wash-out.14 MAC-BAR is the alveolar concentration of anesthetic that blunts adrenergic responses to noxious stimuli. It has been approximated at 50% higher than standard MAC. 15 MAC values also have been established for discreet levels of EEG activity, such as onset of burst suppression or isoelectricity.
Standard MAC values are roughly additive. Administering 0.5 MAC of a potent agent and 0.5 MAC of N2O is equivalent to 1 MAC of potent agent in terms of preventing patient movement, although this does not hold over the entire range of N2O doses. MAC effects for other response parameters are not necessarily additive. For example, combining 0.6 MAC of N2O with 0.6 MAC of isoflurane produces less hypotension than 1.2 MAC of isoflurane alone because isoflurane is a more potent vasodilator and myocardial depressant at equivalent MAC than N2O.
Various factors increase (Table 17-4) or decrease (Table 17-5) MAC. Unfortunately, no single mechanism explains these alterations, supporting the view that anesthesia is the net result of numerous and widely varying physiologic alterations. In general, those factors that increase CNS metabolic activity, neurotransmission, and CNS neurotransmitter levels increase MAC; upregulated CNS responses to chronically depressed neurotransmitter levels (as in chronic alcoholism) also seem to increase MAC. Conversely, those factors that decrease CNS metabolic activity, neurotransmission, and CNS neurotransmitter levels, as well as downregulated CNS responses to chronically elevated neurotransmitter levels all seem to decrease MAC. Many notable factors do not alter MAC, including duration of administration, gender, type of surgical stimulation, thyroid function, hypo- or hypercarbia, metabolic alkalosis, hyperkalemia, and magnesium levels. However, there may be a genetic component influencing MAC. Red-haired females have a 19% increase in MAC compared with dark-haired females.16 These data suggest involvement of mutations of the melanocyte stimulating hormone receptor (MC1R) allele. Variants of the MC1R allele also have been implicated in altering analgesic responses to a κ opioid.17 MAC also can vary in relationship to genotype and chromosomal substitutions as shown in rats.18
Table 17-5. Factors that Decrease Minimum Alveolar Concentration | ||
---|---|---|
|
The Effect of Age on MAC

Other Alterations in Neurophysiology
The modern potent anesthetics, isoflurane, desflurane, and sevoflurane, all have reasonably similar effects on a wide range of parameters including CMRO2, the EEG, cerebral blood flow (CBF), and flow–metabolism coupling. There are notable differences in effects on intracerebral pressure (ICP), cerebrospinal fluid production and resorption, CO2 vasoreactivity, CBF autoregulation, and cerebral protection. Nitrous oxide departs from
the potent agents in several important respects, and is therefore discussed separately.
the potent agents in several important respects, and is therefore discussed separately.
Although neuroprotection from volatile anesthetics is a well-defined concept, the volatile anesthetics can cause injury in certain conditions via cerebral vasodilation and increases in intracranial pressure. A full understanding of the anesthetic effects on cerebral physiology helps prevent adverse cerebral events in clinical practice.
Cerebral Metabolic Rate and Electroencephalogram
For most of the potent agents, CMRO2 is decreased only to the extent that spontaneous cortical neuronal activity (as reflected on the EEG) is decreased. Once this activity is absent (an isoelectric EEG), no further decreases in CMRO2 are generated. (Historically, halothane was the exception.)
Isoflurane causes a larger MAC-dependent depression of CMRO2 than halothane, and because of this, can abolish EEG activity at clinical doses that are usually well tolerated from a hemodynamic standpoint.21 Desflurane and sevoflurane both cause decreases in CMRO2 similar to isoflurane.22,23 Interestingly, while both desflurane and sevoflurane depress the EEG and abolish activity at clinically tolerated doses of approximately 2 MAC22,23 in dogs, desflurane-induced isoelectric EEG reverts to continuous activity with time despite an unchanging MAC, a property unique to desflurane.23
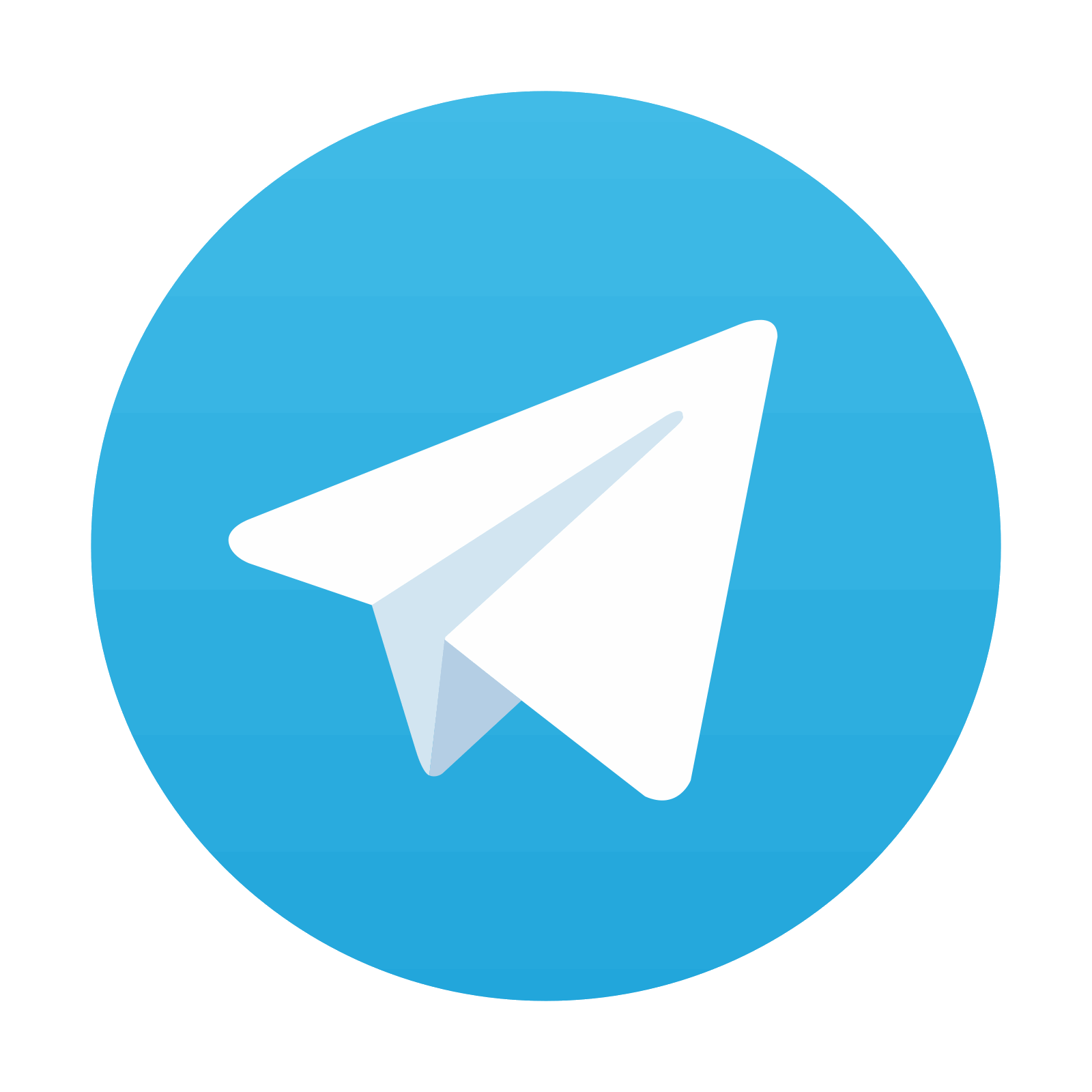
Stay updated, free articles. Join our Telegram channel
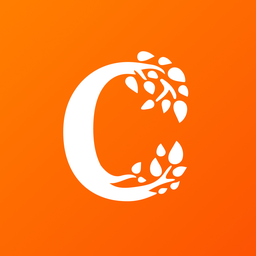
Full access? Get Clinical Tree
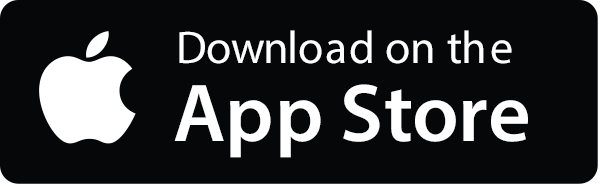
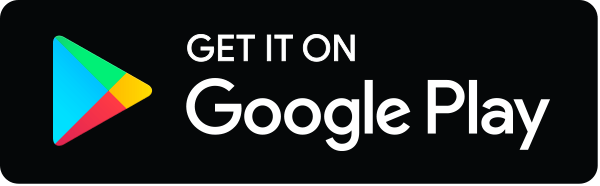
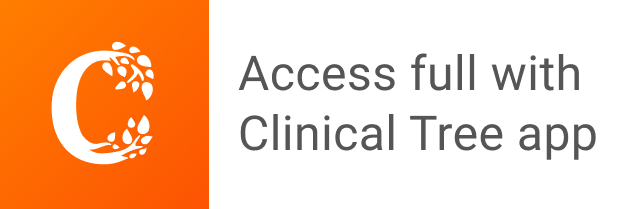