Key Points
The alveolar anesthetic concentration (F A ) or partial pressure (P alv ) is important because it is the driving force determining anesthetic uptake into blood and target tissues in the central nervous system, and it can be monitored as a readout of anesthetic dosage. P alv is influenced by both delivery and uptake of anesthetic gas.
Inhaled anesthetic delivery to patients can be increased with larger fresh carrier gas flows, vaporizer output settings, and minute ventilation.
Initial anesthetic uptake into blood increases with greater pulmonary blood flow (cardiac output) and high blood solubility of anesthetic gas. Increased uptake (as with a highly blood-soluble drug or high cardiac output) slows anesthetic induction because it slows the rate of rise of P alv . Conversely, low anesthetic solubility in blood is associated with rapid onset and offset of anesthesia.
Uptake of anesthetic into blood slows as blood and tissue partial pressures increase, resulting in higher anesthetic partial pressure in mixed venous blood.
The higher the inspired anesthetic concentration, the less it diminishes because of uptake (the concentration effect). At 100% inspired concentration of a gas, uptake reduces the volume of gas in alveoli, but does reduce P alv . During inhalation of anesthetic mixtures containing high nitrous oxide (N 2 O) concentrations, the reduction of alveolar volume produced by rapid N 2 O uptake sustains or increases the concentrations of other alveolar gases (the second gas effect).
Factors that affect anesthetic uptake similarly affect pulmonary clearance of anesthetics. The rate of clearance is also context sensitive—that is, equivalent drops in alveolar and brain anesthetic concentrations are slower after a long exposure to inhaled anesthetic compared to a short exposure of equal depth.
Toxicities of inhaled anesthetics that last beyond the exposure period are primarily associated with their biotransformation (metabolism). These toxic effects are usually produced in the tissues, such as liver and kidney, in which metabolism occurs. Modern inhaled anesthetics undergo less metabolism than older drugs, resulting in less hepatic and renal toxicity.
Halothane hepatitis is a potentially fatal syndrome of fulminant liver damage after exposure to reactive metabolites produced by oxidation of volatile anesthetics. These metabolites covalently modify liver proteins, creating neohaptens that elicit an immune response against hepatocytes. The incidence of the syndrome varies with different anesthetics, paralleling the extent of drug metabolism: halothane >> enflurane > isoflurane > desflurane.
Defluorination of inhaled anesthetics occurs in both the liver and kidney, producing high fluoride concentrations in blood. Renal toxicity characterized by high-output renal failure is almost exclusively associated with prolonged exposure to methoxyflurane. Sevoflurane metabolism also results in high fluoride levels in blood but does not damage kidneys. Factors that enhance the toxicity of methoxyflurane relative to sevoflurane include its higher tissue solubility, slower clearance, and higher degree of renal metabolism, resulting in high intrarenal fluoride levels for an extended time.
In laboratory mammals, including nonhuman primates, all general anesthetics alter synapse and neural circuit formation during critical periods of brain development, resulting in abnormal memory and behavior. Clinical studies in children suggest that lengthy (>4 hours) anesthetic exposures at under 2 years of age are associated with detectable but quite small neurocognitive deficits, relative to unexposed controls (also see Chapter 77 . Postoperative delirium and cognitive decline/dysfunction (POCD) in elderly patients is also a growing concern (see Chapter 83 ). Based on animal and clinical studies, both postsurgical neuroinflammation and exposure to general anesthesia may contribute to POCD.
Anesthetics react with strong bases, particularly potassium hydroxide (KOH), in carbon dioxide (CO 2 ) absorbents, resulting in production of several potentially toxic substances. Sevoflurane degrades to form compound A, which is associated with renal damage in rodents, but not in humans. This differential toxicity is associated with differential renal metabolism in rodents versus humans. Dry CO 2 absorbents in the breathing circuit react with inhaled anesthetics, releasing carbon monoxide and heat. New CO 2 absorbent materials that lack strong base chemicals prevent these reactions and subsequent potential harm to patients.
N 2 O is unique among anesthetics in its ability to inhibit methionine synthase by oxidizing a cofactor, vitamin B 12 . In certain susceptible patients or with multiple frequent exposures to N 2 O, methionine synthase inhibition can lead to hematologic and neurologic dysfunction. After prolonged N 2 O exposure, methionine synthase inhibition also increases blood homocysteine, which is hypothesized to increase vascular inflammation and the risk of thrombosis. Large clinical trials show that N 2 O exposure does not increase the risk of cardiovascular morbidity in most patients, but N 2 O should be avoided in patients with deficiencies in dietary B 12 intake or absorption or B 12 -dependent metabolism.
Inhaled anesthetics, when scavenged and ejected into the atmosphere, contribute to both global warming and ozone depletion. Minimizing the environmental impact of inhaled anesthetics requires reducing waste, through the routine use of low fresh gas flows and/or by use of new technologies that trap scavenged anesthetic gases. Reprocessing and reusing trapped anesthetic gases further reduce the environmental impact of drug production.
Introduction
Modern inhaled anesthetics are important pharmacologic tools for reversibly altering central nervous system (CNS) functions in patients. Because inhaled anesthetics are both taken up and eliminated through alveolar blood-gas exchange, drug dosage can be monitored in expired alveolar gases and tissue-dependent metabolism is unnecessary for drug clearance. Optimal delivery of systemic drugs via inhalation requires a full understanding of the factors influencing how gas-phase compounds move into and out of various body tissues and how they are metabolized (pharmacokinetics) together with knowledge of where and how these drugs and their metabolism affect tissue functions. Reversible anesthetic effects on the nervous, respiratory, and cardiovascular systems (pharmacodynamics) are covered elsewhere in this book (see Chapter 11, Chapter 14, Chapter 19, Chapter 21 ).
Uptake and Distribution of Inhaled Anesthetics
In the first part of this chapter, we review and apply some of the basic principles of chemical equilibria to illuminate major factors influencing inhaled anesthetic uptake and distribution in patients. For this, we use a physiologic model that closely simulates clinical observations. The model, an elaboration of that introduced in 1973 by Mapleson, is described both qualitatively and quantitatively (using mathematical expressions) to convey important concepts to readers with different learning styles.
Biophysical Properties of Inhaled Anesthetics: Partial Pressure, Hydrophobicity, and Partition Coefficients
Inhaled anesthetics are administered as a component of a gas mixture. Biophysical properties of inhaled anesthetics are summarized in Table 20.1 . Partial pressure is the portion of total pressure contributed by one component of a gas mixture, where each component contributes pressure in direct proportion to its molar fraction. For example, 1.5% isoflurane in air (21% O 2 and 79% N 2 ) at 1 standard atmosphere (atm) (760 mm Hg) is a mixture of O 2 at 157.2 mm Hg, N 2 at 591.4 mm Hg, and isoflurane at 11.4 mm Hg. The partial pressure of an anesthetic gas is a measure of its thermodynamic activity and determines its pharmacologic effect. The partial pressure of an anesthetic is usually reported as the percentage (or fraction) of the delivered gas mixture, where atmospheric pressure is near 1 atm (760 mm Hg). Correcting these values to absolute partial pressure is important under conditions when local atmospheric pressure differs significantly from standard, such as at high altitude, underwater, or in a hyperbaric chamber. The same inhaled concentration of an anesthetic gas results in a reduced pharmacologic effect at higher altitudes because the partial pressure of the anesthetic is lower. Because partial pressure is the thermodynamic force for gas transfer between compartments in a system, anesthetics move from regions of high partial pressure to low partial pressure, unaffected by the other components of the gas mixture, and equilibrium is achieved when the partial pressure of an anesthetic is equal in the different compartments.
The maximum partial pressure of a volatile compound is its vapor pressure ; this is the partial pressure of volatile anesthetic (VA) within the drug reservoir of a vaporizer. Vapor pressure is unique to each anesthetic and increases with increasing temperature. VAs are defined by a vapor pressure less than 1 atm at 20°C and a boiling point above 20°C (see Table 20.1 ). Gaseous anesthetics are defined by a vapor pressure above 1 atm at 20°C and a boiling point below 20°C (see Table 20.1 ). VAs typically compose a small fraction of the gas mixture delivered to patients. In contrast, gaseous anesthetics such as nitrous oxide (N 2 O) and xenon, because of their relatively low anesthetic potencies, typically compose a large fraction of an inhaled gas mixture, and thus produce additional effects (e.g., concentration effect, second gas effect, and airspace expansion) that are negligible with VAs.
Hydrophobicity is a molecular property of certain chemicals, including most general anesthetics that do not readily form hydrogen bonds and therefore display low water solubility. Hydrophobic compounds are also usually lipophilic , demonstrating high solubility in low polarity solvents such as oils. Common measures of hydrophobicity are partition coefficients between water and olive oil (which is mostly oleic acid, an 18-carbon fatty acid) or between water and n-octanol. Usually represented by the Greek letter lambda (λ), a partition coefficient is the ratio of two solute concentrations at equilibrium (i.e., at equal partial pressure) in two separate but adjacent solvents or compartments such that the solute moves freely between the compartments ( Fig. 20.1 ). Another useful way to conceptualize a partition coefficient is that it represents the relative volume of two phases or compartments that contain an equal amount of the solute at equilibrium (see Fig. 20.1 ).

Anesthetic partition coefficients between blood and gas (λ b/g ) and between tissue and blood (λ t/b ) are important factors in uptake and distribution of inhaled drugs as they move from pulmonary airspace to pulmonary blood and then from blood to various tissues ( Tables 20.1 and 20.2 ). The blood solubility of anesthetic gases (and other gases such as O 2 , N 2 , and CO 2 ) increases as temperature decreases. Because most anesthetics are hydrophobic, they tend to display high solubility in tissues with high lipid content (e.g., fat), and also bind to many proteins that form hydrophobic or amphiphilic pockets. Anesthetic partitioning into blood (blood solubility) increases after ingestion of fatty foods and may decrease in anemic or malnourished patients. Methoxyflurane (no longer in clinical use) and halothane are notable for high blood solubility. N 2 O, sevoflurane, and desflurane are characterized by low blood solubility.
Tissue | Blood | Heart | Kidney | Liver | CNS | Muscle | Fat | VPT | ||||||||||||||||||||||
---|---|---|---|---|---|---|---|---|---|---|---|---|---|---|---|---|---|---|---|---|---|---|---|---|---|---|---|---|---|---|
Blood Flow (L/min) | Volume (L) | Blood Flow (L/min) | Volume (L) | Blood Flow (L/min) | Volume (L) | Blood Flow (L/min) | Volume (L) | Blood Flow (L/min) | Volume (L) | Blood Flow (L/min) | Volume (L) | Blood Flow (L/min) | Volume (L) | Blood Flow (L/min) | Volume (L) | |||||||||||||||
5 | 5 | 0.2 | 0.28 | 1.07 | 0.32 | 1.2 | 3.9 | 0.62 | 1.43 | 0.75 | 30 | 0.5 | 13 | 0.35 | 7 | |||||||||||||||
Anesthetic Agent | V eff (L) ∗ | λ tissue/blood | V eff (L) | τ (min) | λ tissue/blood | V eff (L) | τ (min) | λ tissue/blood | V eff (L) | τ (min) | λ tissue/blood | V eff (L) | τ (min) | λ tissue/blood | V eff (L) | τ (min) | λ tissue/blood | V eff (L) | τ (min) | λ tissue/blood | V eff (L) | τ (min) | ||||||||
Nitrous oxide | 2.35 | 0.87 | 0.24 | 1.2 | 0.93 | 0.3 | 0.3 | 1.1 | 4.1 | 3.4 | 1.1 | 1.6 | 2.6 | 1.2 | 36 | 48 | 2.3 | 30 | 60 | 1.4 | 9.9 | 29 | ||||||||
Halothane | 12.5 | 2.9 | 0.8 | 4.0 | 1.5 | 0.5 | 0.4 | 2.5 | 9.8 | 8.0 | 2.7 | 3.9 | 3.3 | 2.5 | 75 | 100 | 65 | 840 | 1700 | 2.3 | 16 | 47 | ||||||||
Methoxyflurane | 60 | 1.2 | 0.34 | 1.7 | 2.3 | 0.74 | .69 | 2.5 | 9.8 | 8 | 2 | 2.9 | 4.7 | 1.6 | 48 | 64 | 76 | 980 | 1960 | 1.2 | 8.5 | 25 | ||||||||
Enflurane | 9 | 1.3 | 0.36 | 1.8 | 2.0 | 0.64 | 0.6 | 2.1 | 8.2 | 6.7 | 1.4 | 2.0 | 3.3 | 1.7 | 51 | 68 | 36 | 464 | 930 | 2 | 14 | 41 | ||||||||
Isoflurane | 7 | 1.3 | 0.36 | 1.8 | 2.3 | 0.74 | 0.69 | 2.4 | 9.4 | 7.6 | 1.5 | 2.1 | 3.5 | 2.9 | 87 | 116 | 45 | 580 | 1160 | 2 | 14 | 41 | ||||||||
Desflurane | 2.25 | 1.3 | 0.36 | 1.8 | 1.0 | 0.32 | 0.3 | 1.4 | 5.5 | 4.5 | 1.3 | 1.9 | 3.0 | 2.0 | 60 | 80 | 27 | 350 | 670 | 2 | 14 | 41 | ||||||||
Sevoflurane | 3.25 | 1.3 | 0.36 | 1.8 | 2.3 | 0.74 | 0.69 | 2.4 | 9.4 | 7.7 | 1.7 | 2.4 | 4.0 | 3.1 | 93 | 120 | 48 | 620 | 1240 | 2 | 14 | 41 |
∗ Blood/gas partition coefficients for anesthetic agents are given in Table 20.1.
Anesthetic Delivery, Uptake, and Distribution: a Multicompartmental Model
Delivering an inhaled anesthetic agent to patients is analogous to an intravenous drug infusion with two major differences: (1) entry of drug into the body is via transalveolar exchange from gas to blood, and (2) clearance is mostly via the same route. Thus inhaled anesthetic delivery is dependent on pulmonary ventilation, whereas uptake and clearance of inhaled anesthetics are also dependent on pulmonary perfusion.
Upstream and Downstream Compartments and Anesthetic Transfer: Bulk Flow and Pressure Gradients
Uptake and distribution of inhaled anesthetic can be readily understood as a series of transfer steps from upstream compartments with high partial pressure to downstream compartments with low partial pressure as depicted in Fig. 20.2 . First, drug is transferred from an anesthesia delivery device, typically an anesthesia machine with a vaporizer designed to deliver specified concentrations (in percent atm) of VA agent, into a fresh gas mixture flowing in a breathing circuit. Second, ventilation transfers gases from the circuit to the alveolar airspace in lung. Third, anesthetic moves by transcapillary diffusion into pulmonary venous blood. Fourth, arterial blood distributes anesthetic to various tissues including the primary target tissue, the CNS. Fifth, venous outflow from tissues converges in the pulmonary artery, and sixth, the mixed venous blood passes through alveolar capillaries where it again equilibrates with alveolar gases.

Gas flow from the anesthesia machine into the breathing circuit is unidirectional. Blood circulation is also largely unidirectional. In transfers from the anesthesia machine (the fresh gas outlet) to breathing circuit and then to alveolar airspace, anesthetic flow can simply be understood as exchange from upstream compartments into downstream compartments. In later steps, such as exchange between alveolar gases and pulmonary capillary blood, flow of anesthetic molecules occurs via diffusion between adjacent compartments separated by a permeable membrane. For simplicity, we have not treated blood as a separate compartment in our model. Distribution of anesthetic to and from various tissues involves both bulk transfer via blood flow and diffusive equilibration across capillary membranes. Note that when anesthetic transfer occurs between gas and blood or between blood and tissue, the effective volume of the downstream compartment must be adjusted with the appropriate partition coefficient (see Table 20.2 ).
Rate of Wash-In of the Circuit: Equilibration Between Vaporizer and Circuit
Equipment for controlled delivery of inhaled anesthetic drugs are described elsewhere in this text (see Chapter 22 ). Wash-in of the ventilator breathing circuit represents an example of bulk transfer exchange, wherein the gas in circuit components is replaced by fresh gases emerging from the gas outlet of the anesthesia machine.
Anesthetic Delivery from the Vaporizer: VA delivery (in liters per minute of gaseous drug) from a vaporizer is closely approximated as the product of the delivered anesthetic concentration (fraction = F del or partial pressure at 1 atm = P del ) of the anesthetic in a gas mixture and the fresh gas flow (FGF):
Thus we can readily calculate the volume of delivered gas-phase anesthetic by simply integrating this function over time. In the simplest case where P del and FGF remain constant,
Fresh Gas Wash-In to the Breathing Circuit: The factors that affect the speed at which the gas mixture delivered from the anesthesia machine replaces gases in the breathing circuit (wash-in) are FGF and the breathing circuit volume (V circ ). Consider a typical situation where FGF at the beginning of an anesthetic is 6 L/min, and the gas volume inside the components of a breathing circuit is 6 L. If FGF is doubled to 12 L/min, then wash-in will proceed at twice the rate (halving the time). Conversely, if the V circ doubles to 12 L, then wash-in will proceed at half the rate (doubling the time).
The gas exchange process is independent of the concentration of anesthetic in the circuit, because the exchange is simply through bulk flow and mixing. However, the difference between the delivered concentration and that in the circuit determines the magnitude and direction of net anesthetic gas flow . When the delivered anesthetic partial pressure (P del ) is greater than that in the circuit (P circ ), net anesthetic flow is into the circuit (and subsequently the patient). To remove anesthetic from the circuit, P del must be less than P circ . When there is no concentration gradient (i.e., equal partial pressures), bulk flow exchange may replace all the old anesthetic molecules with new ones, but there is no net flow and anesthetic concentrations in the circuit remain unchanged.
Mathematically, we can describe the breathing circuit exchange process as a differential equation that incorporates all of the above factors:
If P del is constant, integrating this equation results in a single exponential function that defines P circ at any given time following a change in P del at t = 0:
P circ approaches P del following an exponential time course with a time constant of τ = V circ /FGF. Thus if V circ = 6 L and FGF = 6 L/min, the exponential time constant will be 1 minute ( Fig. 20.3 ). Each minute results in the fraction of old gas in the breathing circuit dropping by 63.1%, and after 4 minutes, less than 2% old gas remains. The half-life for the process (time for halving the vaporizer-circuit concentration difference) is 0.693 × τ.

Breathing circuit components, such as CO 2 absorbents and the plastic or rubber of the circuit tubing and connectors, influence the rate of equilibration between vaporizer and circuit, because they absorb VAs, increasing the effective circuit volume. The more hydrophobic VAs absorb more into circuit components, whereas absorption negligibly affects wash-in and wash-out of low-solubility anesthetics.
The clinical relevance of the wash-in process is readily appreciated. An example of the importance of FGF is “priming” the anesthetic circuit for a single-breath induction technique. The FGF setting and the circuit volume influence the required duration of priming. More generally, whenever the vaporizer settings are altered, the speed at which the new settings influence the wash-in or wash-out of the circuit (and subsequently the patient) will depend on FGF. Open (nonrebreathing) anesthetic breathing circuits are designed to have low exchange volumes and to be used with high fresh-gas flows. These features allow rapid changes in the delivered anesthetic concentration, while minimizing rebreathing of exhaled gases. The choice of an open versus rebreathing system influences the impact of various other factors that can affect uptake and distribution of inhaled anesthetics downstream from the breathing circuit. Some of the subsequent figures show models for both conditions.
Equilibration Between Circuit and Pulmonary Airspace
Transfer of anesthetic gases from the breathing circuit to the pulmonary airspace is another bulk exchange process similar to that from vaporizer to breathing circuit. In this case, gas flow via ventilation is cyclical and bidirectional, and the factors that determine the rate of anesthetic exchange are minute ventilation (MV) and total pulmonary airspace volume (V pulm ). Because transfer from the circuit to the lungs represents anesthetic flow out of the circuit, we alter Eq. (20.3) to include both inflow to the circuit and outflow from the circuit,
where P pulm is a weighted average of the anesthetic partial pressure in dead space and alveolar space.
Eq. (20.5) describes how rebreathing affects the inhaled (breathing circuit) anesthetic concentration. Most inhaled anesthetics are delivered using a rebreathing circuit, which includes one-way flow valves and adsorbent material to chemically remove exhaled CO 2 . Rebreathing depends primarily on the balance between fresh gas flow and MV. The anesthetic gas in the breathing circuit represents a mixture of fresh gas and exhaled gases. Increased fresh gas flow reduces rebreathing, whereas increased MV increases rebreathing.
The Alveolar Anesthetic Concentration
The alveolar anesthetic concentration (P alv or F A ) is a critically important factor in anesthetic uptake and distribution because (1) it is in rapid equilibrium with circulating blood and highly perfused tissues, including target tissues in the CNS, and (2) P alv can be measured in exhaled end-tidal gases. Thus, except during periods of rapid change, P alv in exhaled breath represents a useful estimate of the anesthetic concentration in the patient’s CNS and other highly perfused organs.
Because only alveolar gas is relevant to transpulmonary exchange of anesthetic into and out of the body, alveolar ventilation ( <SPAN role=presentation tabIndex=0 id=MathJax-Element-6-Frame class=MathJax style="POSITION: relative" data-mathml='V˙alv’>V˙alvV˙alv V ˙ alv ) is the proper gas flow to calculate anesthetic exchange into this part of the pulmonary airspace,
where <SPAN role=presentation tabIndex=0 id=MathJax-Element-8-Frame class=MathJax style="POSITION: relative" data-mathml='V˙alv’>V˙alvV˙alv V ˙ alv is MV corrected for dead space ventilation.
Alveolar Uptake of Anesthetic into Pulmonary Blood
During inhaled anesthetic induction, anesthetic flows from alveolar gas to pulmonary blood across the alveolar/capillary interface separating these compartments and is driven by the partial pressure gradient between alveolar gas (P alv ) and mixed venous blood (P MV ) entering the pulmonary arteries. The net flow of anesthetic reverses during anesthetic wash-out when P alv drops below P MV . Anesthetic uptake into blood also depends on the pulmonary blood flow (which is typically close to cardiac output, <SPAN role=presentation tabIndex=0 id=MathJax-Element-9-Frame class=MathJax style="POSITION: relative" data-mathml='Q˙’>Q˙Q˙ Q ˙ ) and the blood’s capacity to solvate anesthetic from the gas state (the blood/gas partition coefficient, λ b/g ):
We therefore correct Eq. (20.6) to reflect both anesthetic inflow into alveolar airspace and its uptake into blood:
Thus, during an inhaled anesthetic induction, the rate of increase of P alv relative to P circ is governed by (1) alveolar ventilation, (2) cardiac output, and (3) anesthetic solubility in blood. Increased ventilation delivers more anesthetic from circuit to alveoli and increases P alv /P circ ( Fig. 20.4 ). Importantly, increased pulmonary blood flow removes more anesthetic from alveoli, thereby decreasing the rate of increase in alveolar concentration of anesthetic (P alv /P circ ) ( Fig. 20.5 ). Indeed, significant decreases in cardiac output are suspected when end-tidal CO 2 (ETCO 2 ) decreases and end-tidal concentration of VA increases. The more soluble an anesthetic is in blood (i.e., the higher its λ b/g ), the greater is the capacity for each volume of blood to take up anesthetic from alveolar gases (i.e., the larger the effective blood flow). Thus as λ b/g increases, P alv /P circ increases more slowly ( Fig. 20.6 ).



Other Factors That Affect the Rate of Rise of P alv
Other factors affecting alveolar uptake of anesthetic include ventilation-perfusion matching and the absolute concentration of anesthetic in alveolar gases.
Pulmonary Dead Space. Dead space (ventilated but not perfused pulmonary regions) reduces effective alveolar ventilation (see Eqs. 20.7 and 20.8 ), and thus slows anesthetic uptake. This effect is strongest under conditions of high FGF and low blood-solubility agents, where alveolar ventilation is the limiting factor in uptake. Under conditions of low FGF and a highly blood-soluble agent, increased dead space, by reducing initial uptake, serves to maintain the inhaled anesthetic concentration (P circ ), which creates a compensatory increase in P alv and subsequent uptake.
Pulmonary (Right to Left) Shunting. Pulmonary right to left shunting can be physiologic, pathologic, or iatrogenic, such as during one-lung ventilation. Right-to-left shunting results in a difference between P alv and the partial pressure of anesthetic in arterial blood (P art ). This is because arterial blood represents a mixture of shunted mixed venous blood combined with blood that has equilibrated with alveolar gases ( Eq. 20.9 ). Because such shunts also reduce transcapillary gas exchange in the lung and slow anesthetic uptake ( Eqs. 20.7 and 20.8 , after correcting pulmonary blood flow for shunt), right-to-left shunting sustains P circ , an effect that is more pronounced for highly soluble drugs compared with insoluble anesthetics. Thus shunt reduces the ratio of P art alv more for insoluble anesthetics, such as N 2 O ( Fig. 20.7 ):

Concentration and Second Gas Effects. The absolute concentration of anesthetic influences its uptake and that of other gases. In the previous discussion and illustrations, it was presumed that an inhaled anesthetic represents a small fraction of the inhaled gas mixture and that transalveolar uptake of the anesthetic results in a decrease in P alv and negligible changes in alveolar gas volume. However, when the anesthetic represents a large fraction of the inhaled gas mixture, its rapid uptake results in a smaller relative alveolar anesthetic concentration drop, because the volume of alveolar gas also decreases. This is known as the concentration effec t. In an imaginary situation where a patient is breathing 100% anesthetic, uptake into pulmonary blood reduces the volume of anesthetic gas in the alveoli without altering its concentration or partial pressure (oxygen-induced atelectasis occurs through a similar mechanism). A typical situation, illustrated in Fig. 20.8 , is delivery of 66% N 2 O with 33% O 2 and 1% isoflurane. Assuming cardiac output equals 5 L/min, the initial rate of N 2 O uptake is given by Eq. (20.7) as 5000 mL/min × 0.47 × 0.66 atm = 1550 mL/min, indicating that a large fraction of N 2 O is taken up into blood during the first few breaths. If we assume that half the N 2 O and half the isoflurane are rapidly taken up following the first breath of this gas mixture, then alveolar volume drops by 33.5% and the remaining alveolar gas contains 33 parts N 2 O, 33 parts O 2 , and 0.5 parts isoflurane (49.6% N 2 O, 49.6% O 2 , and 0.8% isoflurane). Despite 50% uptake of N 2 O, the significant reduction in alveolar gas volume results in a concentration of remaining alveolar N 2 O that is only 24% less than its initial value.

The second gas effect is also evident in this example: the rapid uptake of N 2 O and reduced alveolar gas volume sustains P iso near its original inspired value and increases alveolar <SPAN role=presentation tabIndex=0 id=MathJax-Element-13-Frame class=MathJax style="POSITION: relative" data-mathml='PO2′>PO2PO2 P O 2 , thereby augmenting uptake of these gases. The rapid uptake of N 2 O into blood also results in an effective increase in MV, because more gas from the breathing circuit is passively drawn into lung as alveolar gas is rapidly absorbed. These effects have been demonstrated in humans and lab animals. Recent clinical studies and mathematical modeling indicate that, because of ventilation-perfusion heterogeneity, the second gas effect is greater in arterial blood than in expired gas, influenced by the blood solubility of VAs, and significantly affects anesthetic onset at relatively low rates of N 2 O uptake.
Distribution of Anesthetic into Tissues
Blood exiting the pulmonary capillaries enters the pulmonary vein and the left heart. Inhaled anesthetics are then distributed via arterial blood to various body tissues. The rate of increase of anesthetic partial pressure within each tissue is determined by tissue-specific arterial blood flow ( <SPAN role=presentation tabIndex=0 id=MathJax-Element-14-Frame class=MathJax style="POSITION: relative" data-mathml='q˙’>q˙q˙ q ˙ ), effective volume (the product of anatomic volume and tissue/blood partition coefficient, <SPAN role=presentation tabIndex=0 id=MathJax-Element-15-Frame class=MathJax style="POSITION: relative" data-mathml='λt/b’>λt/bλt/b λ t/b ), and the anesthetic partial pressure gradient between arterial blood and the tissue,
where i designates a particular organ or type of tissue. Values used in model calculations are summarized in Table 20.2 . The time required for anesthetic partial pressure equilibration between arterial blood (P art = P alv ) and a specified tissue is shorter if its blood flow is high, and longer if that tissue has a large effective volume ( Figs. 20.2 and 20.9 ).

Traditionally, anesthetic distribution has been described for four distinct tissue groups. The vessel-rich group (VRG) includes the heart, brain, lungs, spinal cord, liver, and kidney. Together, these organs compose approximately 10% of the adult human body mass; however, they receive approximately 70% of cardiac output under normal resting conditions. As a result, time constants for anesthetic equilibration between blood and these organs are typically only a few minutes (see Table 20.2 ). Of particular interest is the equilibration time for the CNS, where anesthetic effects are mediated. After the highly perfused VRG tissues, skeletal muscle is the next compartment to equilibrate with inhaled anesthetics. Muscle composes approximately 40% of body mass in a healthy adult, making muscle the largest single compartment based on weight. Moreover, most inhaled anesthetics partition into muscle more than into brain, resulting in an increased effective volume for anesthetic uptake into this compartment. At rest, muscle receives about 10% to 15% of cardiac output (20 mL/kg/min), but this value can increase dramatically during exercise, stress, fever, or other states associated with high cardiac output. Taken together, these factors generally result in slow equilibration between anesthetic in blood and muscle, with typical time constants of hours (see Table 20.2 ). The third tissue group is fat , which in a healthy adult composes less than 25% of body mass and receives approximately 10% of cardiac output. Potent VAs partition avidly into fat; therefore, fat represents the largest effective volume for uptake of these drugs (see Fig. 20.2 , Table 20.2 ). The extremely large effective volume coupled with low blood flow results in very slow equilibration of anesthetics between blood and fat, with time constants approaching days. A fourth group, including skin, cortical bone, and connective tissue, is referred to as vessel poor tissues . These tissues compose 10% to 15% of an average adult body while receiving less than 5% of cardiac output at rest. Induction of general anesthesia impairs normal sympathetic nervous function, resulting in increased blood flow to normally cool skin in the extremities. The blood volume represents approximately 7% of body mass and may be considered another compartment for anesthetic uptake, while also conveying drug to other tissue compartments.
As stated previously, increased cardiac output results in increased anesthetic uptake and a slower rate of rise of P alv . Clinical studies confirm that, other factors being equal, increasing cardiac output slows the induction of general anesthesia with inhaled anesthetics. This result can seem counterintuitive when increasing cardiac output increases uptake of anesthetic into the patient’s body and hastens its delivery to the tissues. However, during induction, the anesthetic partial pressure in blood and downstream tissue compartments cannot be higher than that in the upstream alveolar compartment. Increased cardiac output slows the rise of P alv and thus also slows the rate of increase of the anesthetic partial pressure in blood (P art ), the CNS (P CNS ), and other highly perfused tissues. The extra anesthetic uptake is primarily into muscle, which is a large tissue compartment with a high capacity for anesthetic and is where much of the excess cardiac output flows. For example, a 50% increase in cardiac output can more than double muscle blood flow, diverting the majority of anesthetic to muscle, lowering P alv , and thus slowing anesthetic uptake into target tissues in the CNS. If one could manipulate inhaled anesthetic delivery to maintain constant P alv , which may be achievable with automated feedback control of vaporizer output and FGF, then increased cardiac output might have a different effect. Model simulations where P alv is maintained at a constant level show that uptake into VRG tissues, including brain tissue, increases more rapidly as cardiac output increases.
In pediatric patients, the balance of cardiac output to various tissue beds differs from that in adults. Thus, although cardiac output per kilogram body weight is larger in children than in adults, anesthetic induction in young children is more rapid than in adults, because a disproportionate amount of perfusion goes to the vessel rich organs, such as the brain.
The equilibrium distribution volumes for most inhaled anesthetics are extremely large, with the largest compartment by far being fat. However, equilibration with fat is so slow that this compartment usually plays a relatively minor role in the pharmacokinetics of inhaled anesthetics. During a typical general anesthetic lasting from 30 minutes to several hours, the blood, VRG organs, and muscle are the compartments into which inhaled anesthetics mostly distribute.
Although the model in Fig. 20.2 illustrates anesthetic distribution only via arterial blood flow, intertissue diffusion takes place between abutting tissues that have large interfacial surface areas. In particular, direct diffusion from organs with high anesthetic partial pressures to abutting tissues with low partial pressure and high capacity for anesthetic uptake may also contribute to drug distribution. Examples of this process include anesthetic diffusion from the heart, liver, and kidneys to surrounding fat in the pericardium and abdomen.
The Mixed Venous Anesthetic Partial Pressure
The anesthetic partial pressure in mixed venous blood entering the pulmonary circulation is a weighted average of the venous outflows from all tissues and organs, which converge in the right ventricle:
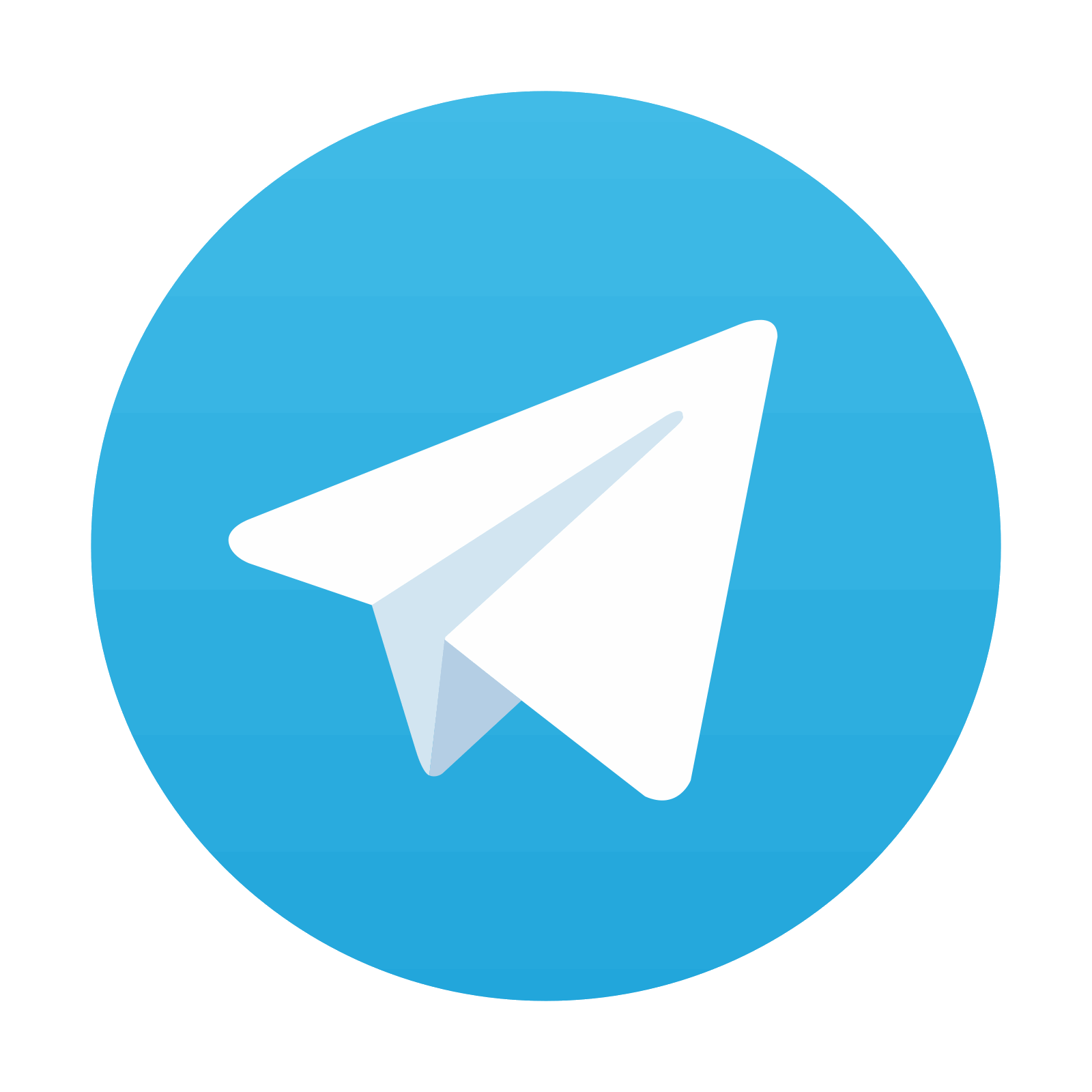
Stay updated, free articles. Join our Telegram channel
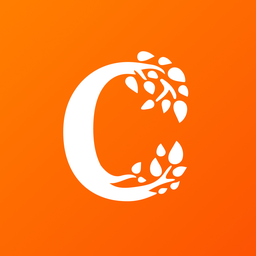
Full access? Get Clinical Tree
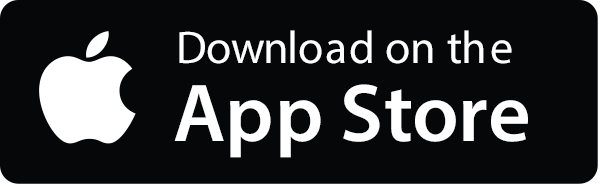
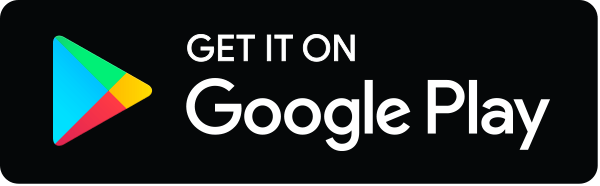