Indirect Calorimetry
Nicholas A. Smyrnios
Frederick J. Curley
Indirect calorimetry is a technique that uses measurements of inspired and expired gas flows, volumes, and concentrations to measure oxygen consumption and carbon dioxide production. Energy expenditure, respiratory quotient (RQ), and other values can be derived from the measured values. Indirect calorimetry provides a noninvasive, accurate method of measuring caloric requirements and oxygen consumption. This chapter focuses on the technique of performing indirect calorimetry in the intensive care unit (ICU) setting. Indirect calorimetry is the subject of a clinical practice guideline that was revised in 2004 by the American Association of Respiratory Care [1].
Theoretical Basis of Indirect Calorimetry
Indirect calorimetry systems rely on measurements of inhaled and exhaled airflow, volume, and concentrations of oxygen and carbon dioxide. Indirect calorimetry systems can be classified as open-circuit systems, which measure the difference between inspired and expired gas concentrations, or closed-circuit systems, which measure changes in the amount of gases in a fixed reservoir over time [2]. This chapter reviews only open-circuit systems because closed circuit systems are rarely used in critical care.
Definitions
Chemical energy used to fuel the human body is directly provided by adenosine triphosphate, which is formed by the oxidation of carbohydrate, protein, and lipid. The stores of adenosine triphosphate in the body are small, but they are in a constant, high-volume, well-balanced state of formation and utilization. Indirect calorimetry measures the oxygen used and carbon dioxide produced when carbohydrate, protein, and lipid are oxidized to produce adenosine triphosphate. Therefore, it is the production of chemical energy that is indirectly measured by the gas-exchange parameters. Despite this, it is the convention to describe this quantity as energy expended. Therefore, we use the term energy expenditure to describe the amount of energy quantified by indirect calorimetry.
In any discussion of energy expenditure, it is important to define what level of energy expenditure is being considered. Basal metabolic rate or basal energy expenditure (BEE) is the energy used by the body at complete rest and in the postabsorptive state (absence of active nutritional intake for at least 4 to 6 hours). This measurement can be obtained reliably only in deep sleep. If such a measurement is made, BEE does take into account the effects of illness and stress. However, BEE often is taken to be a value calculated from a standardized equation that does not account for stress, such as the Harris-Benedict equation. Resting energy expenditure (REE) is obtained from an awake person at rest and includes the energy used at rest in the awake state plus the energy used to metabolize foodstuffs, also called diet-induced thermogenesis. It is expected to be approximately 10% greater than BEE [3, 4 and 5]. Total energy expenditure (TEE) is REE plus the energy used during activity. TEE and REE are closer in value for ICU patients than for patients in other settings because most ICU patients are bedridden. In most cases, we wish to know the 24-hour REE or TEE rather than the BEE.
Calculation of Energy Expenditure
Most indirect calorimetry systems use the modified de Weir equation to calculate energy expenditure. In its more complete form, this equation uses oxygen consumption ([V with dot above]o2), carbon dioxide production ([V with dot above]CO2), and nonprotein urinary nitrogen (UN):
Energy expenditure = 3.9([V with dot above]o2) – ([V with dot above]CO2) – 2.17(UN g/day)
The de Weir equation is not experimentally derived from measurements on humans but is mathematically derived. The derivation relies on the knowledge that (a) TEE is equal to the sum of the energy expended from the combustion of carbohydrate, fat, and protein; (b) the caloric equivalents of glucose (3.7 kcal per g), fat (9.5 kcal per g), and protein (4.1 kcal per g) are known; (c) the oxygen consumed and carbon dioxide produced in metabolizing each of these fuels is known; and (d) therefore, the equation for energy expenditure can be expressed in terms of oxygen consumption and carbon dioxide production by solving the system of equations that describes the stoichiometry of fuel combustion. The reader is referred to other sources for a complete derivation of the equation [6]. In practice, the amount of urea nitrogen in the urine is usually not measured because of the fact that its contribution to TEE is considered minimal.
Calculation of Oxygen Consumption
The essential measurements of indirect calorimetry are the inspired and expired oxygen fractions (Fio2 and FEO2, respectively), carbon dioxide fractions, (FICO2 and FEO2, respectively) and minute ventilation. Oxygen consumption and carbon dioxide production can be calculated using similar equations, which
compute the difference between inspired (I) and expired (E) volumes:
compute the difference between inspired (I) and expired (E) volumes:
Oxygen consumption = [V with dot above]o2 = VI(Fio2) – [V with dot above]E(FEO2
Carbon dioxide production = [V with dot above]CO2 = [V with dot above]E(FEco2) – [V with dot above]I(FICO2)
To avoid the need to measure the volumes of expiratory and inspiratory gases, techniques have been developed that preferentially measure only exhaled volumes and mathematically derive the inhaled volume. Any assumption that the volume exhaled is equal to the volume inhaled is erroneous whenever [V with dot above]CO2 and [V with dot above]o2 are not equal (i.e., RQ is not equal to 1) and becomes more erroneous as Fio2 increases. A mathematic relationship of [V with dot above]E to [V with dot above]I called the Haldane transformation can be used to explain this phenomenon. It takes advantage of the fact that nitrogen is an essentially inert gas. Therefore,
Volume inspired ([V with dot above]I) × FEN2 = volume expired ([V with dot above]E) × FEN2
Rearranged, this reads
[V with dot above]I = [V with dot above]E × FEN2/FIN2
Because FIO2 FIN2 = 1 and FEO2 + FECO2 + FEN2 = 1, FIN2 = 1 – FIO2
and
FEN2 = 1 – FECO2 – FEO2
If we substitute back into the previous equation,
[V with dot above]I = [V with dot above]E × (1 – FECO2 – FEO2)/(1 – FIO2)
As the inspired oxygen concentration increases, the denominator decreases and the difference between inspired and expired gas volume becomes greater. The Haldane equation can therefore be used to determine the value of [V with dot above]I without measuring inspired volume.
The use of the Haldane equation during indirect calorimetry leads to some important and practical technical considerations. Most significantly, the accuracy of the Haldane equation in estimating inspired volume, and thereby oxygen consumption and energy expenditure, depends greatly on the accuracy of measurement of FIO2 and [V with dot above]E. Any error in measuring exhaled volumes or gas concentrations directly produces an error of a greater magnitude in the calculation of oxygen consumption, carbon dioxide production, and energy expenditure. At a minute ventilation of 10 L per minute and an inspired-to-expired oxygen concentration difference of 0.03, the oxygen consumption would be 300 mL per minute. If the FIO2 was measured to be 0.46 instead of 0.45 and the FEO2 remained at 0.42, the oxygen consumption would be 400 mL per minute, a 33% change. If the measured minute ventilation was 10.1 L per minute and the actual value was 10 L per minute, there would be an error in the oxygen consumption of 30 mL per minute, or 10%. To prevent such errors, the analysis system must be free of leaks, and extremely accurate sensors must be used. Because most oxygen sensors are less accurate at higher FIO2, indirect calorimetry studies are usually limited to patients on 60% oxygen or less. Although some systems have provided accurate results in vitro with higher levels of oxygen, there have been no studies to date that prove the accuracy of any system above FiO2 60% in critically ill patients [7, 8]. The 2004 American Association of Respiratory Care guideline also confirms the limitation of FEO2 to 0.6 [1].
Equipment and Technique
Open-circuit indirect calorimetry systems all involve certain basic components, typically an oxygen analyzer, a carbon dioxide analyzer, and a flowmeter (usually a pneumotach). Most systems also use masks, canopies, mixing chambers, tubing, desiccants, and pumps. Some newer systems developed for use on ambulatory patients are small enough to be held in one hand. These ambulatory devices are not validated for use on critically ill patients.
Methods of Measurement
Oxygen sensors in commercially available systems are either zirconium or differential paramagnetic sensors. The zirconium oxide sensor is coated with an oxygen-permeable substance. At temperatures of approximately 800°C, oxygen diffuses across this outer layer and an electrical signal that is proportional to the partial pressure of oxygen is created [2, 9]. Differential paramagnetic analyzers measure the difference in concentration of the gas between the inspiratory and expiratory lines. These analyzers typically have an accuracy of ± 0.02% and a response time of 130 msec or less. Essentially all available carbon dioxide analyzers are nondispersed infrared devices. A gas sample in the path of infrared energy creates an alteration in an electrical signal that is proportional to the concentration of carbon dioxide. These analyzers have an accuracy of ± 0.02% with a response time of 110 msec. Some systems measure inspired and expired carbon dioxide, and some measure only expired, assuming the inspired value to be negligible. Volume is calculated by measuring flow and integrating the result over time to obtain volume. Flow is usually either measured with a pneumotach or a mass flow sensor or generated by the device and kept constant in response to changes in ventilation.
Gas concentrations are measured using one of three techniques: mixing chamber, breath-by-breath, and dilution. All devices must have well-validated calibration procedures for essential components. Newer machines have automated much of this process.
Mixing Chamber Method
The mixing chamber is the best-established method and has been considered the gold standard. A mixing chamber is an automated Douglas bag that mixes expired gases over a predetermined interval and provides the material to be sampled [9]. Expired gas is passed from a mouthpiece or the ventilator exhalation port into a collection chamber, which is in series with the flowmeter and gas analyzers. Inside the chamber, baffles interrupt airflow to create a more even mixing of gases. A sample of mixed gas is withdrawn from the chamber, the gas concentrations are analyzed, and the sample is returned to the chamber. Depending on the design, the gas is either vented or passed through the flowmeter. The concentrations of inspiratory gas are sampled from the inspiratory side of a mouthpiece or a ventilator circuit. Inspiratory volumes are calculated from expiratory volumes as explained previously. A computer compares mixed expired versus inspired concentrations and multiplies by volume to yield a measure of consumption or production. The results reflect the values of gases mixed over time and are reported as values per time interval of measurement (e.g., milliliters of oxygen consumed per minute).
Breath-by-Breath Method
The collection and analysis of gases in the breath-by-breath method are similar to those in the mixing chamber technique, but each breath is analyzed. A sample of gases is taken for analysis from each inspiration and expiration. These samples are coupled with flow measurements for each breath to calculate [V with dot above]o2, [V with dot above]CO2, and REE. The concentrations of expiratory gases are measured directly from samples drawn from the expiratory side of the mouthpiece or ventilator circuit. Inspired concentrations are measured from samples drawn from the inspiratory side of a mouthpiece or ventilator circuit. Oxygen consumption and carbon dioxide production values are usually expressed as milliliters per minute and energy expenditure as kilocalories per day for each breath and can be averaged or summed over varying periods, depending on the clinician’s needs. The crucial component in these measurements is the alignment of various signals. If the time needed for the gases to reach the analyzer and the expiratory flow to reach the flowmeter is known, the [V with dot above]o2, [V with dot above]CO2, and [V with dot above]E signals can be precisely aligned and accurate measurements made. Instruments that use breath-by-breath analysis align the signals automatically by computer. Improper alignment can render the measurements useless. Oxygen and carbon dioxide sensors must have a very rapid response time. The placement of the gas analysis line just distal to the endotracheal tube can standardize transit time and eliminate artifact, which assists in this process.
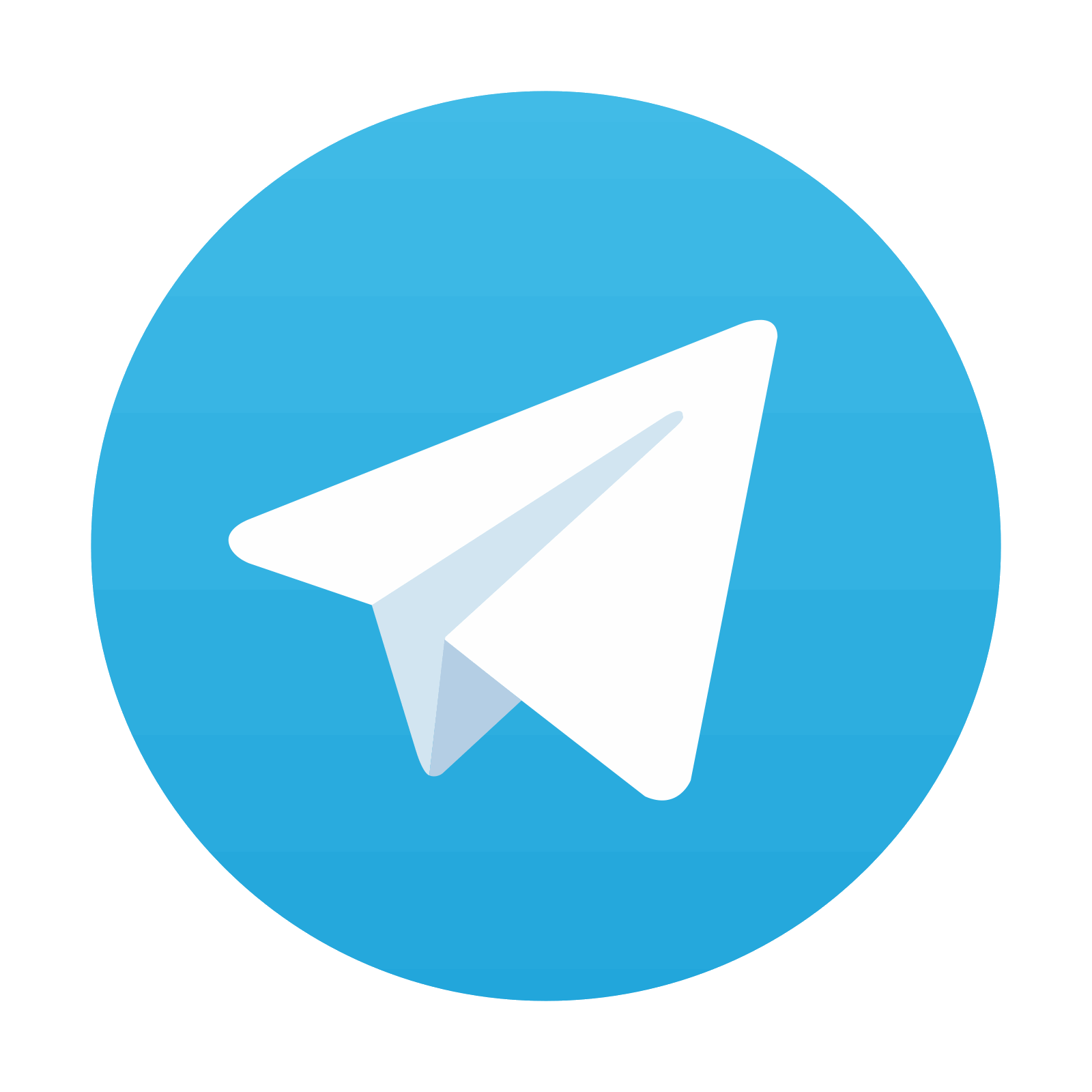
Stay updated, free articles. Join our Telegram channel
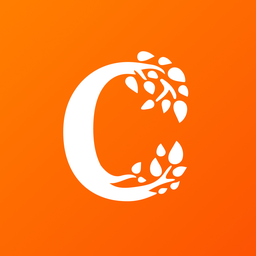
Full access? Get Clinical Tree
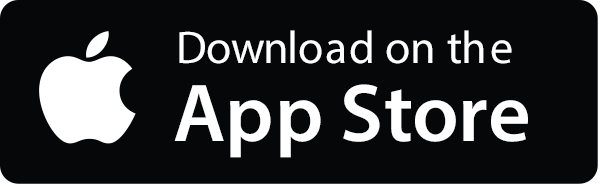
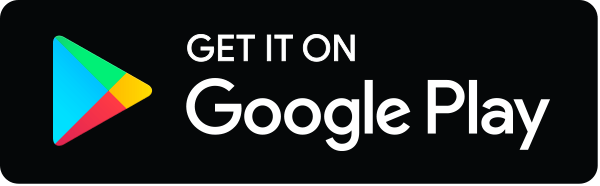
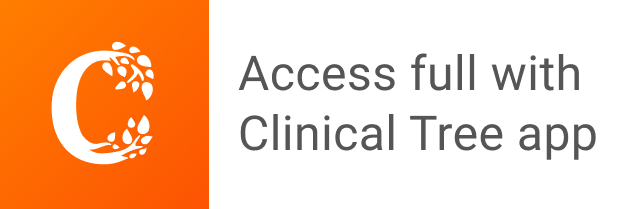