Hypoxia occurs when tissues do not receive adequate oxygen to meet their metabolic demands. It is nonspecific and can mean reduced arterial partial pressure of oxygen (PaO2; hypoxemic hypoxia), reduced hemoglobin for oxygen transportation (anemic hypoxia), decreased cardiac output or regional decrease in perfusion (stagnant hypoxia), disarray in cellular metabolism of oxygen such as in cyanide (histotoxic hypoxia), or mitochondrial dysfunction in sepsis (cytopathic dysoxia).1
Type 1 respiratory failure is defined as PaO2 less than 60 mmHg with an equivalent arterial oxygen saturation (SaO2) of 90% and normal or near or low arterial partial pressure of carbon dioxide (PaCO2). Type 2 respiratory failure involves hypercapnia and is defined as a PaCO2 of 46 mmHg or higher, regardless of oxygen saturation. Type 1 respiratory failure will be discussed in this chapter.
Two percent of oxygen is dissolved in plasma (PaO2) and 98% is bounded to hemoglobin (SaO2). One gram of hemoglobin (Hgb) carries 1.34 mL O2 per gram. Oxygen delivery is based on the formula DO2 = CaO2 × CO, where DO2 is the diffusing capacity of oxygen, CaO2 is the arterial concentration of oxygen, and CO is cardiac output. Oxygen content is derived from the formula CO2 = (1.39 × Hgb × SaO2/100) + (0.003 × PO2), where CO2 is the concentration of oxygen, Hgb is the hemoglobin, SaO2 is fraction of oxygen saturated hemoglobin over total hemoglobin, and PO2 is the partial pressure of oxygen. Oxygen consumption is derived from the formula V.O2 = CO × (CaO2 – CvO2), where CvO2 is the venous concentration of oxygen. The alveolar–arterial (A-a) oxygen gradient is PAO2 – PaO2, where PAO2 = (FiO2 × [PATM – PH2O]) – (PaCO2/R). In these formulas, PAO2 is the alveolar oxygen tension; PaO2 is the arterial pressure of oxygen; FiO2 is the fraction of inspired oxygen, which is 0.21 on room air; PATM is atmospheric pressure, which is 760 mmHg at sea level, PH2O is the partial pressure of water, which is 47 mmHg at 37°C; PaCO2 is arterial carbon dioxide tension; and R is 0.8 at steady state. The normal A-a gradient varies with age and is based on the formula 2.5 + 0.21 × age in years.
A common, noninvasive way to measure oxygen bounded to hemoglobin is oxygen saturation via pulse oximetry. Based on the Beer-Lambert law, the principle states that light absorption of a given wavelength through a nonabsorbing solvent is directly proportional to the solute concentration, light path length, and extinct coefficient.2 Deoxyhemoglobin absorbs light at a maximum of 600 to 750 nm, so light at 660 nm is optimal for its detection, and oxyhemoglobin absorbs light at a maximum of 850 to 1000 nm, so light at 940 nm is optimal for its detection.3,4 In carbon monoxide poisoning, carboxyhemoglobin absorbs light at 660 nm and may cause a falsely normal or high O2 saturation reading.5,6 In methemoglobinemia, methemoglobin absorbs light at both 660 and 940 nm and may cause falsely low O2 saturation.7,8
Another consideration is that the correlation of peripheral arterial oxygen saturation (SpO2) with SaO2 worsens when SpO2 is less than 90%. Pulse oximetry also cannot detect hyperoxemia because large changes in PO2 results in no change in oxygen saturation if it is almost 100% (Fig. 8-1).
Hypoxemia refers to a decreased PaO2. The 5 mechanisms of hypoxemia include reduced oxygen tension, hypoventilation, V̇/Q̇ mismatch, shunt, and diffusion abnormalities.9,10 For a particular disease, these mechanisms are not necessarily mutually exclusive.
The partial pressure of inspired oxygen (PiO2), depends on FiO2, PATM in mmHg, and PH2O in mmHg according to the following equation: PiO2 = FiO2 × (PATM – PH2O). For a person breathing room air at sea level and 37°C, the equation is PiO2 = 0.21 × (760 – 47) = 150 mmHg. This equation demonstrates that as the FiO2 increases, the PiO2 increases. Furthermore, as atmospheric pressure decreases (as it does at higher altitudes), the PiO2 is lower. In the absence of diffusion abnormalities, the PaO2 is determined via the PiO2. If the PiO2 is low enough, it will result in hypoxemia. Therefore, a decreased FiO2 and increased altitude (lower PATM) can cause hypoxemia (decreased PaO2). For example, for a person breathing room air at 37°C on Mount Everest (~29,500 ft, PATM = 231 mmHg) the equation is PiO2 = 0.21 × (231 – 47) = 39 mmHg, and for a person breathing a 100% FiO2 at sea level and 37°C, the equation is PiO2 = 1.00 × (760 – 47) = 713 mmHg.
Inspired air is made up mostly of nitrogen (N2) and O2. When this air reaches the alveoli, it has mixed with water from the airways and CO2 that was not expired on the last breath. The approximate partial pressures of gases in alveolar air are as follows: PO2 = 100 mmHg, PCO2 = 40 mmHg, PH2O = 47 mmHg, and PN2 = 573 mmHg, for a total alveolar pressure of 760 mmHg. In the setting of hypoventilation, total alveolar pressure remains constant but the partial pressures change. Incomplete exhalation leads to poor clearance of CO2 from the alveoli, but diffusion of CO2 from blood to alveolus continues as long as a gradient still remains. The result is an increased partial pressure of CO2 in the alveolus. Because the sum of all alveolar gases must remain constant, an increased partial pressure of CO2 results in a decreased PAO2. Decreased PAO2 results in a lower PAO2–PaO2 gradient, causing decreased diffusion of oxygen across the alveolar-capillary membrane and a decreased PaO2. Because the respiratory quotient is 0.8 (CO2 produced/O2 consumed), hypoventilation affects CO2 levels more than O2 levels.
A V̇/Q̇ mismatch refers to ventilation and perfusion that are disproportionate to one another. In the ideal lung, ventilation would match the perfusion (V̇/Q̇ = 1) in all parts of the lung. However, even in healthy individuals, there is a small amount of V̇/Q̇ mismatch due to gravity and airflow resistance. Both ventilation and perfusion are increased in the bases of the lungs, but perfusion is affected more than ventilation is. The result is increased perfusion compared to ventilation (V̇/Q̇ < 1) in the lower portions of the lungs and increased ventilation compared to perfusion (V̇/Q̇ > 1) in the upper portions of the lungs. Diseased lungs have a greater degree of V̇/Q̇ mismatch due to areas of decreased ventilation, areas of decreased perfusion, or both.
In the scenario of high ventilation and low perfusion (V̇/Q̇ > 1), supplemental oxygen will improve hypoxemia because increased FiO2 will increase the oxygen gradient from the alveolus to the blood and aid in diffusion. The extreme of this scenario, with ventilation but no perfusion (V̇/Q̇ = ∞) is called dead space. An example of dead space is the trachea, where ventilation is high but there is no pulmonary circulation to participate in gas exchange. There is both anatomic dead space and physiologic dead space. Anatomic dead space includes the oropharynx, trachea, and other non–gas exchanging airways, and is estimated at about 150 ccin a normal adult but varies based on height and anatomy. Physiologic dead space is pathologic and refers to a diseased area of the lung with normal (or increased) ventilation but no perfusion.
The extreme of the scenario of low ventilation and high perfusion (V̇/Q̇ < 1), with no ventilation and normal (or increased) perfusion, is shunt and will be discussed in further detail later.
Shunt refers to blood flowing from the right to the left heart without participating in gas exchange. The result is deoxygenated blood returning to systemic circulation. If enough shunt is present, hypoxemia will result. Shunt fraction can be calculated using the following equation: Qs/Qt = (CcO2 – CaO2) / (CCO2 – CvO2). Where Qs/Qt is the shunt fraction, CcO2 is the end-capillary oxygen content, CaO2 is the arterial oxygen content, and CvO2 is the mixed venous oxygen content. Shunt is an extreme form of V̇/Q̇ mismatch in which perfusion is present without ventilation (V̇/Q̇ = 0). In its true form, supplemental oxygenation does not improve hypoxemia because there is no ventilation to deliver the oxygen to the alveolar-capillary membrane. Shunt can be either structural or physiologic. Structural shunts include a patent foramen ovale (PFO) or ventricular septal defect (VSD) with Eisenmenger syndrome. Physiologic shunts include pulmonary arteriovenous malformation (AVM) or alveolar-filling processes such as dense consolidation due to pneumonia or atelectasis.
Blood is oxygenated by diffusion of oxygen from the alveoli to the pulmonary capillaries. This diffusion is based on an oxygen gradient with higher concentrations of oxygen in the inspired alveolar air and lower concentration of oxygen in the venous blood returning to the lung. While carbon dioxide diffusion occurs very rapidly, oxygen diffusion requires a longer time to complete. Even so, a normal alveolar-capillary unit has enough surface area such that the blood passing by requires only about one-third of its contact time to fully equilibrate. When the alveolus or interstitium is disrupted by inflammation or fibrosis, the time for oxygen equilibration lengthens. With enough compromise of the alveolar-capillary membrane, oxygen diffusion time will lengthen to the point where full equilibration is not achieved by the time the blood has traversed the alveolus, resulting in hypoxemia. Hypoxemia due to diffusion abnormalities may be detected earlier in patients when testing includes pulse oximetry monitoring during exertion because as cardiac output increases, blood transit time across the alveolus decreases.
Target oxygen saturation is 94% to 98%, but the elderly may have oxygen saturation less than 94% and remain clinically stable.1 Nevertheless, exercise desaturation of 5% or more may be perceived as abnormal despite maintaining these target goals. Patients at risk for hypercapnia should have an oxygen saturation of 88% to 92%.1 The method of oxygen supplementation is changed to meet these goals. Initial oxygen supplementation may be nasal cannula at 2 to 6 L/min, face mask at 5 to 10 L/min, or reservoir mask if SO2 less than 85, venturi mask 28% at 4 L/min for patients at risk for hypercapnia, or nonrebreather.1
A nasal cannula can deliver up to 6 L/min of oxygen. Flow higher than 6 L/min might increase patient discomfort or dryness, and increase the risk of epistaxis. It works primarily by washing out dead space in the nasal and oropharynx, thus increasing the oxygen content and decreasing the carbon dioxide content of inspired air. For every 1 L/min of oxygen delivered via nasal cannula, FiO2 increases by 3% to 4%. Thus, a nasal cannula can deliver a maximum FiO2 of about 45%. However, if the patient has limitations of airflow via the nasal passages or is breathing primarily through the mouth, the delivered FiO2 will be lower than expected. In addition, if the patient is breathing rapidly, dead space washout will be suboptimal and the delivered FiO2 will be lower.
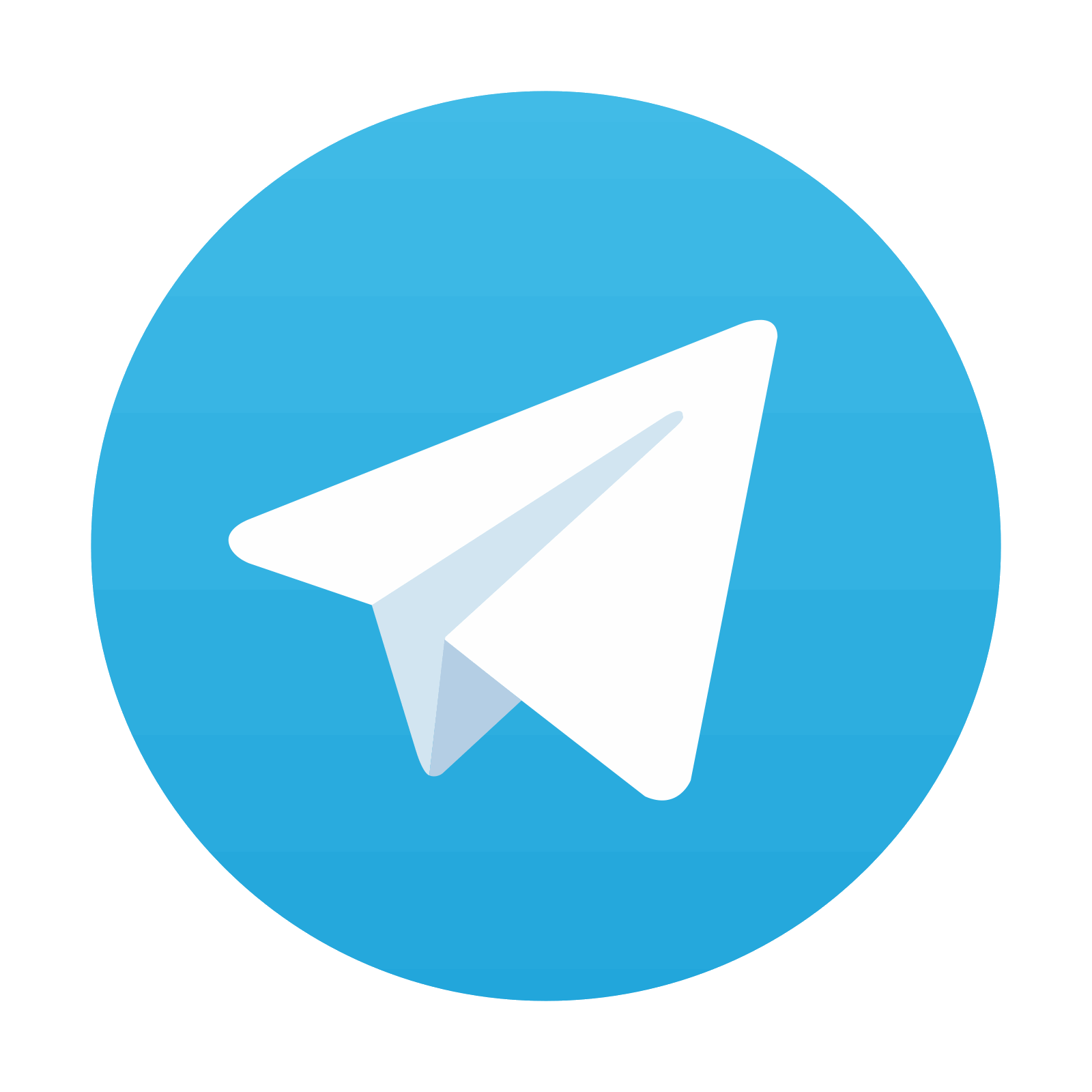
Stay updated, free articles. Join our Telegram channel
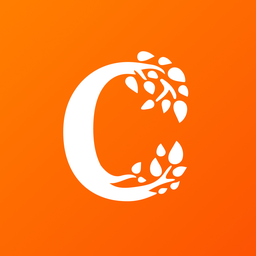
Full access? Get Clinical Tree
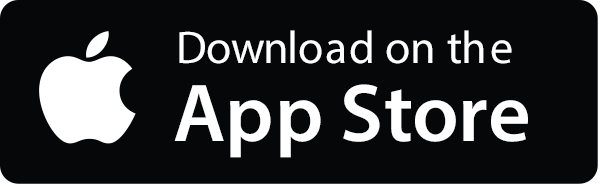
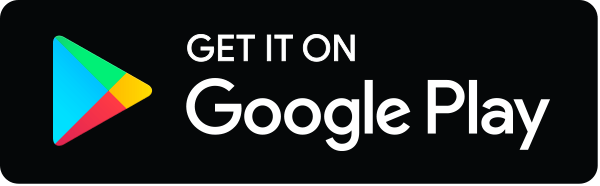