Chapter 30
Hepatobiliary and Gastrointestinal Disturbances and Anesthesia
The hepatobiliary system plays an integral role in anesthetic management. An intimate familiarity with diseases of the hepatobiliary and gastrointestinal systems can help avoid negative anesthetic outcomes. The liver is the largest internal organ and is critical in maintaining the homeostasis of many other physiologic systems, the least of which is drug metabolism. As such, acute or chronic liver dysfunction can impair the intended response to anesthesia.1
Liver Disease
Anatomic, Physiologic, and Pathophysiologic Considerations
Located in the thoracic portion of the abdominal cavity, the liver is the largest organ in the body and generally extends from rib 7 to rib 11 along the right midaxillary line. The liver spans from the right hypochondrium to a portion of the left hypochondrium and is classically divided into four lobes, which may be subdivided into multiple segments as described, for example, by the Couinaud system.2 These subdivisions are based on the anatomic proximity of hepatic and portal veins, though multiple classifications are used that contain different terminology and no system is recognized as superior.
The functional unit of the liver is the hepatic lobule or acinus. The acinus forms around a portal canal consisting of a portal venule, hepatic arteriole, bile ductule, lymphatic vessel, and nerves. The acinus architecture radiates around a central vein that empties into the hepatic veins and then into the vena cava (Figure 30-1). Hepatic lobules number between 50,000 and 100,000 in the normal liver.
The filtering function of the liver has a prominent physiologic role. Blood from the gut contains large quantities of colonic bacilli; it is cleansed of more than 99% of the bacterial load by Kupffer cells (macrophages) that line the hepatic sinuses.3 Endothelial cells that line the hepatic sinuses permit diffusion of large plasma proteins and other substances into the extravascular spaces in the liver. This phenomenon results in a large quantity of lymph that is nearly equal in protein concentration to plasma.
The metabolic functions of the liver require a significant quantity of blood, which comes from arterial and venous sources. The portal vein and the hepatic artery provide the primary blood supply to the liver, delivering approximately 1.5 liters per minute of blood flow. The hepatic artery branches off of the abdominal aorta and delivers 400 to 500 mL/minute of oxygenated blood. According to the anatomic peculiarity of the double afferent blood supply of the liver, 75% to 80% of the blood entering the liver is partially deoxygenated venous blood supplied by the portal vein, which collects all the blood that leaves the spleen, stomach, small and large intestine, gallbladder, and pancreas. The blood entering the liver via the hepatic portal vein contains some oxygen and is high in nutrients that have been absorbed from the digestive tract into the mesenteric and portal veins. Hepatic venous blood supply is shown in Figure 30-2. It is responsible for 70% of blood flow to the normal liver (≈1 L/minute), but only 50% of the liver oxygen supply. The dual supply of blood allows the liver to be relatively resistant to hypoxemia. The combined blood from both sources joins in the hepatic sinusoidal channels lying between the layers of cells in the lobule. These channels serve as capillaries. Endothelial cells and Kupffer cells line the sinusoids. Bile canaliculi are located between hepatocytes; these canaliculi empty into terminal bile ducts. A coalescence of central veins from hepatic lobules forms the hepatic veins, which empty into the inferior vena cava. An extensive arcade of lymphatic vessels is also present within the layer of cells.4
The mean pressure in the hepatic artery is similar to that in the aorta, whereas portal vein pressure has been reported to range between 6 and 10 mmHg in humans. This relatively low pressure allows the liver to function as a circulatory reservoir. Hepatic blood volume may expand considerably in cardiac failure and, in turn, serves as an important blood reservoir in case of bleeding episodes, and compensates up to 25% of the hemorrhage by immediate expulsion of blood from the capacitance vessels.5 Both α- and β-receptors are present in the hepatic arterial circulation, but only α-receptors are noted in the portal circulation. There is disagreement as to whether the hepatic arterial vasculature exhibits autoregulation of blood flow. Portal blood flow is dependent on the combined venous outflow from the spleen and gastrointestinal tract. A decrease in either portal or arterial blood flow affects a compensatory increase in blood flow delivered by the other system.6
Potent inhaled anesthetics can affect hepatic blood flow, particularly portal blood flow, but present evidence suggests that flow is well maintained relative to oxygen demand. None of the present anesthetics adversely influence hepatic integrity by its effects on blood flow.7 Effects of volatile anesthetics on hepatic blood flow are described in Table 30-1.
TABLE 30-1
Effect of Selected Volatile Anesthetics on Hepatic Blood Flow
Volatile Anesthetic | Hepatic Artery Blood Flow | Portal Vein Blood Flow |
Desflurane | No change | Decrease or no change |
Isoflurane | Increase | No change |
Sevoflurane | No change or increase | Decrease |
Changes in hepatic artery or portal vein blood flow may not result in an overall change in total hepatic flow due to the hepatic artery buffer response (HABR). This response is a semireciprocal autoregulatory mechanism whereby changes in portal flow inversely affect hepatic arterial flow.8 The similarity in total hepatic flow between agents implies an intact HABR.
The liver has many physiologic functions, including synthesis and metabolism of various essential proteins, lipids, and hormones (Box 30-1). There are no artificial devices that can duplicate all of the functions of the liver.
Protein Synthesis
Protein synthesis occurs primarily in the liver; this excludes immunoglobulins, which are produced by the humoral immune system. With significant liver disease, a reduction in circulating plasma protein will result in a decrease in plasma oncotic pressure. Additionally, drugs bound to proteins produced by the liver would have a greater unbound fraction if circulating proteins were reduced due to liver disease. In addition, overexpansion of the interstitial space and third-spacing secondary to derangements in plasma oncotic pressure result in a large increase in the volume of distribution of clinically used medications. Clinical concerns should therefore focus on the potential for an exaggerated effect with a given dose of drug, particularly a drug that is highly protein bound. The amount of nondepolarizing muscle relaxant may also need to be increased to achieve a given level of blockade. This is secondary to an increased volume of distribution of the drug (secondary to alterations in plasma protein binding and body fluid shifts). Plasma cholinesterase, which is produced in the liver, also may be deficient. This condition may prolong the effects of succinylcholine as well as enhance the potential toxicity of ester local anesthetics.
Bile Production
A deficiency in vitamin K results in coagulopathy due to impaired production of clotting factors II (prothrombin), VII, IX, and X.9 Except for factor VIII, which is produced in endothelial cells, the liver is responsible for producing all clotting factors. Hepatocellular disease therefore results in decreased clotting factor levels and abnormal bile production. Impaired bile production ultimately manifests as altered production of vitamin K–dependent clotting factors.
Insulin Clearance
The liver is the main site for insulin clearance, removing 50% during the first portal passage, but this percentage varies widely under different conditions.10 During obesity, hyperinsulinemia, insulin-resistant state, dyslipidemia, and type II diabetes mellitus insulin clearance in the liver decreases. A reduction in hepatic insulin extraction would lead, in insulin-resistant states, to a substantial peripheral hyperinsulinemia (due to insulin hypersecretion and reduced hepatic extraction of insulin).11
Drug Metabolism/Transformation
The enzyme systems involved in the biotransformation of drugs are located primarily in the liver. Proper hepatic function is necessary to maintain the pharmacokinetic machinery detailed earlier in this textbook. Orally administered drugs may be metabolically inactivated in the liver before reaching the systemic circulation. This first-pass metabolism may limit the oral availability of highly metabolized drugs. Within the liver, phase I and phase II reactions are responsible for metabolism of many exogenous substances and most drugs. The subsequent products are then excreted via excretory transporters on either the canalicular or sinusoidal membranes12 (Box 30-2). The end products of these processes (except in the case of prodrugs or an active metabolite) are the result of deactivation and transformation of substances into benign by-products capable of being excreted in the bile or urine.
The cytochrome P450 (CYP) class of enzymes are primarily responsible for phase I reactions. More than 50 CYPs have been identified in humans, yet nearly 50% of drugs currently manufactured are metabolized by CYP 3A4/5.13 Differences in the rate of metabolism of a drug can be due to drug interactions. When two drugs are coadministered and subjected to metabolism by the same enzyme system, the rate of metabolism can be either decreased or increased. Enzyme induction hastens metabolism of certain coadministered medications (e.g., ethanol, barbiturates, ketamine, some benzodiazepines) and promotes tolerance to other medications metabolized by the same enzyme class. This relative tolerance can increase the clinical requirement for other drugs (e.g., sedatives, opioids, steroid muscle relaxants). Conversely, coadministration of drugs metabolized by a single CYP (e.g., cimetidine, chloramphenicol) will compete for binding to the enzyme’s active site. This can result in enzyme inhibition of metabolism of one or both of the drugs and lead to elevated plasma levels culminating in increased sensitivity or toxicity.
Laboratory Evaluation of Liver Function
Ammonia is cleared by the liver and may be used to evaluate hepatic encephalopathy, but ammonia levels do not correlate well with severity of the clinical presentation. As such, its usefulness is limited. Bilirubin levels also reflect hepatic clearance effectiveness, but it is elevated in most significant liver diseases and is not specific in diagnostic value. Albumin synthesis occurs in the liver, and hypoalbuminemia can indicate chronic liver disease once nonhepatic etiologies have been ruled out. Because albumin has a half-life of 14 to 21 days, quantitative laboratory analysis will be slow to decrease in relation to worsening liver function, making it an unreliable indicator of hepatic synthetic function in acute liver injury. The most common reason for a low albumin is chronic liver failure caused by cirrhosis. The serum albumin concentration is usually normal in chronic liver disease until cirrhosis and significant liver damage has occurred. In advanced liver disease, the serum albumin level may be less than 3.5 g/dL. Biochemical markers of liver function are identified in Table 30-2.
TABLE 30-2
Clinical Significance of Liver Biochemical Tests
∗The normal values tabulated are for adult men and will vary with the methodology used in testing.
Patients who present soon after passing common bile duct stones can be misdiagnosed with acute hepatitis because aminotransferase levels often rise immediately, but alkaline phosphatase and γ-glutamyl transferase levels do not become elevated for several days. Asymptomatic patients with isolated, mild elevation of either the unconjugated bilirubin or the γ-glutamyl transferase value usually do not have liver disease and generally do not require extensive evaluation.14
Overall hepatic function can be assessed by applying the values for albumin, bilirubin, and prothrombin time in the Child-Pugh classification system, which is modified from the earlier Child-Turcotte grading system15 (Table 30-3).
Effects of Anesthesia on Liver Function
Patients with liver disease who require surgery are at greater risk for surgical- and anesthesia-related complications than those with a healthy liver.16 The degree of the risk is dependent on the anesthetic technique and associated sequelae, the surgery being performed, and specific type of liver disease and its severity.
Volatile Anesthetic Selection
All of the volatile anesthetics also have been shown to reduce hepatic blood flow. Halothane causes the greatest reduction, and the use of desflurane has been shown to have hepatic effects similar to those of isoflurane. A rise in serum glutathione-S-transferase (GST) level indicates a decrease in splanchnic circulation, which causes a transient reduction in the oxygenation of hepatocytes. Anesthesia with desflurane has demonstrated that liver function is well preserved.17 Sevoflurane undergoes hepatic biotransformation, producing organic and inorganic fluoride ion. In human subjects, levels of serum inorganic fluoride ion secondary to sevoflurane metabolism are generally below nephrotoxic levels. Prolonged use of higher concentrations, however, may lead to problematic levels. Anesthetic agents may reduce hepatic blood flow by 30% to 50% after induction. Animal data suggest, however, that isoflurane (along with desflurane and sevoflurane, which are believed to be similar) causes less perturbation in hepatic arterial blood flow than other inhaled anesthetic agents and therefore is preferred for patients with liver disease. Studies continue with sevoflurane to determine the influence of biotransformation on renal and hepatic function, but no significant clinical toxicity has yet been reported and they appear to be safe in clinical use.18,19
In developed countries, clinical use of halothane as a volatile anesthetic agent is being superseded by the newer low-solubility agents desflurane and sevoflurane. Halothane, however, is still in use in some countries and remains on the 2011 World Health Organization Model List of Essential Medicines, which is a list of minimum medical needs for a basic health care system. Continued awareness of the potentially deleterious effect of halothane on hepatic function is therefore justified.
Halothane administration is associated with two types of postoperative liver injury. Minor injury in 10% to 30% of patients may result in elevations in alanine aminotransferase (ALT) levels during postoperative days 1 through 10. Risk of hepatotoxicity is higher after repeat exposure to halothane. Major injury involves halothane-induced hepatotoxicity, which is a severe hepatic reaction with elements of autoimmune allergy. The phenomenon of halothane-induced liver damage has been termed halothane hepatitis due to a similar clinical presentation. Clinical features of halothane hepatitis are listed in Box 30-3. Hepatic necrosis may be seen histologically, and the case fatality rate ranged from 14% to 71% (before liver transplant was an option). Evidence for the role of hypersensitivity is found in the increased susceptibility and shortened latency after repeat exposure, the hallmark symptoms and signs of drug allergy. Risk factors for halothane hepatitis are listed in Box 30-4. An estimated 1 in 10,000 patients develops postoperative jaundice after halothane exposure. In this population, a viral source of infection is more likely to be the cause—for instance, as a complication of intraoperative blood transfusion.
Opioid Effects
Spasm of the Oddi sphincter may cause biliary colic, or it may cause a false-positive result on intraoperative cholangiography. All opioids have been implicated in causing spasm of the Oddi sphincter, with a resultant increase in biliary pressure in relation to potency. The cause of the spasm is unclear, but occurrence may be reduced with judicious titration. Spasm is low even when a fentanyl-based anesthetic is used. The treatment of suspected spasm of the Oddi sphincter involves administration of naloxone or nalbuphine. Atropine or glycopyrrolate, glucagon, and nitroglycerin also have been shown to be effective.
Anesthesia-Related Activity: Mechanical Ventilation
The sequelae of mechanical ventilation have been implicated as a contributing factor in a reduction of hepatic blood flow. Positive pressure ventilation can result in airway pressures that adversely affect venous delivery to the right atrium. Increased airway pressures also result in reduced cardiac output,20 with a consequent reduction in hepatic blood flow. Positive end-expiratory pressure further exacerbates this condition. Impairment in hepatic blood flow under these conditions may result from increased hepatic venous pressure from increased intrathoracic pressure and from increased reflex sympathetic tone caused by reduced cardiac output. Hypercapnia and acidosis have vasodilatory effects on the hepatic circulation that result in increased blood flow, whereas hypocapnia and alkalosis exert vasoconstricting effects that result in decreased flow. The interplay of various intraoperative variables (e.g., surgical site, ventilatory mode, direct and indirect effects of anesthetics used, physiologic responses to intraoperative events) influences the degree of variation in hepatic blood flow.
Site of Surgery
Surgical site, particularly intraabdominal, also has been implicated as a cause of decreased hepatic blood flow (see Table 30-4 for reported surgery risk in patients with liver disease). Traction on the abdominal viscera may cause reflex dilation of splanchnic capacitance vessels and thereby lower hepatic blood flow.
Additional factors that may contribute to decreased hepatic blood flow intraoperatively include hypotension, hemorrhage, vasoactive drugs, and pneumoperitoneum during laparoscopic surgery.21
Diseases of the Liver
Viral Hepatitis
Hepatitis is a generic term that means liver inflammation. Hepatitis may be caused by several factors (e.g., toxins, alcohol, medications, viral or bacterial infections, autoimmune diseases), and viral hepatitis is the leading cause of liver cancer and the most common reason for transplantation.22
Although several types of hepatitis viruses are known to cause illness, typically only hepatitis A, B, and C (infections caused by the hepatitis A virus [HAV], hepatitis B virus [HBV], and hepatitis C virus [HCV], respectively) affect persons living in the United States.22 Hepatitis D requires co-infection with HBV; hepatitis E virus (HEV) diagnosis is made by exclusion after travel to an endemic area (e.g., South/Central America or Southeast Asia). Hepatitis A and E are transmitted by the oral-fecal route, and hepatitis B, C, and D are transmitted by contact with body fluids and physical contact with disrupted cutaneous barriers.
The common clinical course of viral hepatitis begins with a 1- to 2-week prodromal period, the signs and symptoms of which include fever, malaise, and nausea and vomiting. Progression to jaundice typically occurs, with resolution within 2 to 12 weeks. However, serum transaminase levels often remain increased for up to 4 months. If hepatitis B or C is the cause, the clinical course is often more prolonged and complicated. Cholestasis may manifest in certain cases. Fulminant hepatic necrosis in certain individuals is also possible. Table 30-5 lists the major characteristics of hepatitis types A, B, C, D, and E.
TABLE 30-5
Five Types of Acute Viral Hepatitis
From Goldman L, Schafer AI, eds. Goldman’s Cecil Medicine. 24th ed. Philadelphia: Saunders; 2012.
Drug-Induced Hepatitis
Drug-related injury to the liver results from an idiosyncratic reaction to a substance or an overdose resulting in toxicity. For idiosyncratic reactions, genetic predisposition is presumed to be the most critical determinant. Important risk factors include age, gender, exposure to other substances, a history or family history of previous drug reaction, other risk factors for liver disease, and concomitant medical conditions.23 Factors related to the risk of liver injury are summarized in Table 30-6.
TABLE 30-6
Factors Influencing the Risk of Liver Diseases Caused by Drugs
From Teoh NC, et al. Liver disease caused by drugs. In: Feldman M, et al, eds. Sleisenger and Fordtran’s Gastrointestinal and Liver Disease. 9th ed. Philadelphia: Saunders; 2010:1416.
Alcoholic hepatitis is probably the most common form of drug-induced hepatitis and results in fatty infiltration of the liver (causing hepatomegaly), with impairment in hepatic oxidation of fatty acids, lipoprotein synthesis and secretion, and fatty acid esterification.6
Chronic Hepatitis
Chronic hepatitis occurs in 1% to 10% of acute hepatitis B infections and in 10% to 40% of hepatitis C infections,6 but does not occur in hepatitis A infections.
Anesthetic Management for Patients with Hepatitis
Evidence from studies indicates that mild chronic hepatitis confers no additional risk of surgical morbidity or mortality during laparoscopic cholecystectomy.24 Surgical outcomes in patients with acute hepatitis are less well studied, and recommendations suggest that elective surgery should be postponed until normalization of biochemical profiles. Existing studies are many decades old and the statistical risks associated may not reflect improvements in either surgical technique or anesthetic management. Anesthetic management recommendations are found in Box 30-5.
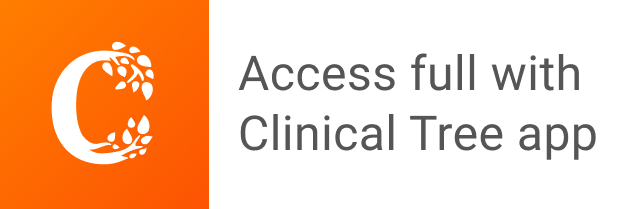