Key Points
- ▪
The liver is the largest abdominal organ and carries out a number of vital functions including metabolism and detoxification.
- ▪
The liver receives approximately 25% of the resting cardiac output. The hepatic artery is responsible for 25% to 30% of the blood supply to the liver while the portal vein is responsible for 70% to 75%. They each deliver 50% of the total oxygen to the liver.
- ▪
For the purpose of hepatic resection, the liver can be divided into eight segments based on independent blood supply and drainage of blood and bile. A segment can be resected without compromising the blood flow and biliary drainage of other segments.
- ▪
The acinus is the basic functional unit of the liver. It is organized around the flow of blood from the portal triad to the central vein through the sinusoids. Hepatocytes in the acinus are organized into zones based on their proximity to the portal triad or central vein. Zone 1 or periportal hepatocytes are closer to the portal triads and receive oxygen and nutrient-rich blood. Zone 3 or perivenous hepatocytes are closer to the central veins and receive oxygen-poor blood. Hepatocytes in different zones serve different anatomic functions.
- ▪
The liver plays an integral role in carbohydrate, protein, lipid, and bile metabolism. It is also responsible for protein synthesis. Albumin is the most abundant plasma protein produced by the liver.
- ▪
Drug and toxin excretion is carried out by the hepatocytes by first polarizing the molecules then conjugating them to make them more hydrophilic. Drugs excreted in the bile may be reabsorbed through enterohepatic circulation leading to prolonged effects.
- ▪
Standard laboratory panels used to evaluate the hepatobiliary system help define broad categories of hepatobiliary pathology: hepatitis, hepatobiliary dysfunction, or insufficient protein synthesis. Specific diagnoses often require clinical context and radiologic studies.
- ▪
Cirrhosis is the result of chronic hepatic disease and can ultimately result in portal hypertension and liver failure. Liver failure can lead to significant dysfunction in all organ systems, giving rise to coagulopathy, thrombocytopenia, hyperdynamic circulation, esophageal varices, hepatic encephalopathy, hepatopulmonary syndrome, portopulmonary hypertension, and hepatorenal syndrome. The definitive treatment for hepatic failure is liver transplantation.
- ▪
Volatile anesthetics reduce mean arterial pressure and cardiac output, leading to a reduction in portal blood flow in a dose-dependent manner. The hepatic arterial buffer response is preserved with isoflurane, sevoflurane, and desflurane leading to the preservation total hepatic blood flow, but not with halothane.
- ▪
Advanced liver disease impairs the elimination of many drugs including vecuronium, rocuronium, morphine, meperidine, and benzodiazepines. Dosing should be adjusted in the setting of liver failure.
- ▪
Elective surgery is contraindicated in patients with acute hepatitis or liver failure. Patients with chronic hepatitis can safely undergo elective surgery. Hepatotoxic drugs should be avoided and hepatic perfusion maintained. Child-Turcotte-Pugh class and Model for End-Stage Liver Disease score can be used to predict risk of perioperative mortality.
Acknowledgment
The editors and publisher would like to thank Drs. Phillip S. Mushlin and Simon Gelman for contributing a chapter on this topic in the prior edition of this work. It has served as the foundation for the current chapter.
Anatomy of the Liver
The liver is the second largest organ in the human body and is responsible for a host of functions to maintain homeostasis. The liver acts as the interface between the gastrointestinal tract and remainder of the body. It is responsible for metabolic, synthetic, immunologic, and hemodynamic functions. As a result, hepatic dysfunction has profound effects on all organ systems and introduces significant challenges to anesthetic management. It is therefore essential for the anesthesiologist to have a firm grasp of the anatomy, physiology, and pathophysiology of the liver.
Surgical Anatomy, Hepatic Blood Flow, and the Biliary Tree
The adult liver can range from around 600 to over 1800 g making the liver one of the heaviest organs in the body. In healthy females the liver ranges in size from 603 to 1767 g, while in healthy males, the liver ranges in size from 968 to 1860 g. In newborns, infants, and children, the liver is also one of the largest organs and the contribution of its weight to total body weight decreases with age. Thus the liver in a term 3 to 3.5 kg newborn can weigh 150 to 170 g, which represents around 5% of the total body weight. In sharp contrast the adult liver represents 2% to 2.5% of the total body weight.
The liver receives approximately 25% of the resting cardiac output (CO). The blood supply is through both the arterial and venous systems in the form of the hepatic artery and the portal vein ( Fig. 16.1 ). The hepatic artery is responsible for 25% to 30% of the blood supply to the liver whereas the portal vein is responsible for 70% to 75%. The hepatic artery arises from the celiac trunk in 80% of the population. In the remainder, it arises from the superior mesenteric artery. After giving rise to the gastroduodenal artery, the common hepatic artery enters the hilum of the liver (porta hepatis) where it further branches into the right and left hepatic arteries, supplying the right and left sides of the liver, respectively. The right hepatic artery gives rise to the cystic artery that supplies the gallbladder. The arteries continue to branch throughout the liver ultimately running through the portal tracts and terminating in the hepatic sinusoids (capillaries). Although part of the venous system, the portal vein is the primary source of oxygenated blood to the liver. The portal vein carries blood from the gastrointestinal tract, pancreas, and spleen to the liver. It drains the superior mesenteric, splenic, and inferior mesenteric veins. It also drains the gastric, cystic, and pancreaticoduodenal veins. The portal vein enters the hilum and, like the hepatic artery, branches into the right and left portal veins, supplying the respective sides of the liver. The portal veins continue to branch throughout the liver in conjunction with the hepatic arteries. As with the arteries, they terminate in the hepatic sinusoids.

Venous drainage of the liver is through the hepatic veins directly into the inferior vena cava (IVC). The right and middle hepatic veins serve the right half and middle portions of the liver, respectively, while the left hepatic vein drains the left half of the liver. The biliary system removes bile from the liver and delivers it to the duodenum through the ampulla of Vater. The intrahepatic bile ducts typically travel with the portal veins, draining into right and left collections systems that ultimately form the common bile duct (CBD; see Fig. 16.1 ).
From a historical perspective, the description of the gross anatomy of the liver has evolved from being rooted in the surface anatomy of the organ to its functional organization. Traditionally, the liver was divided into four lobes based on its surface features: right lobe, left lobe, quadrate, and caudate. The right and left lobes were divided by the falciform ligament, when viewed anteriorly. When viewed from below, the quadrate lobe was bounded by the porta hepatis posteriorly, the gallbladder fossa on the right, and the ligamentum teres on the left. The caudate lobe was bounded by the porta hepatis anteriorly, the IVC on the right, and the ligamentum venosum fissure on the left. In the late 1800s, Sir James Cantlie demonstrated that the right and left hemilivers were defined by independent portal circulations and thus the functional midline of the liver was at the bifurcation of the portal vein, along a line connecting the gallbladder bed and the IVC (“Cantlie’s line”), lateral to the falciform ligament. Cantlie recognized that the line defined a vascular watershed and described its implications for surgical resection of the liver. With advances in hepatic surgery, anatomic descriptions of the liver were developed that further divided the hemilivers into segments based on the vascular distribution and biliary drainage. Each segment has its own independent vascular inflow and outflow, and biliary drainage. As a result, surgical resection of a segment does not compromise adjacent segments. The most commonly used organizational system was developed by Couinaud (see Fig. 16.1 ). In the Couinaud model, the liver is divided into eight segments. The right and left hemilivers are divided at the bifurcation of the portal vein, along the middle hepatic vein. The right, middle, and left hepatic veins divide the liver vertically into four sectors: right posterior, right anterior, left medial, and left lateral sectors. The four sectors are divided in the horizontal plane by the branches of the portal vein, giving rise to the eight segments. In this system, the caudate lobe is referred to as segment 1 and the remainder of the segments are numbered in a clockwise fashion. Segments 2 and 3 are medial to the left hepatic vein, with segment 2 superior to segment 3. Segment 4 lies between the middle and left hepatic vein and is subdivided into 4a (superior) and 4b (inferior) subsegments. Segments 8 (superior) and 5 (inferior) are located between the middle and right hepatic veins. Segments 6 (inferior) and 7 (superior) are located between the right hepatic vein and the edge of the liver. In clinical practice, contrast-enhanced computed tomography (CT) scanning and intraoperative ultrasound are used to define the anatomy unique to each individual and plan for the appropriate resection. To standardize the nomenclature used to describe hepatic resections, the International Hepatopancreatobiliary Association published consensus terminology in 2000 based on the Couinaud segments, known as the Brisbane 2000 terminology. This system of terminology has gained traction but has not yet been uniformly adopted.
Cellular Anatomy
Liver Lobule and Acinus
The cellular architecture of the liver supports its functions of detoxifying the blood and metabolizing nutrients. Histologically, the liver parenchyma can be organized into anatomic units (liver lobules) or functional units (liver acinus). The liver lobule is the basic structural unit of the hepatic parenchyma ( Fig. 16.2 ). The lobule typically appears hexagonal in shape with a portal canal at each corner and a hepatic venule (central vein) located in the center. Through each portal canal run the lymphatics, nerve fibers, and a portal triad. Each portal triad consists of a bile ductule, hepatic arteriole, and portal venule. From a functional standpoint, the acinus is the smallest unit of the liver ( Fig. 16.3A ). It is comprised of a portal tract at the center with central vein at the periphery. Oxygenated and nutrient-rich blood flows from the portal triads to the central hepatic veins through the hepatic sinusoids (see Fig. 16.3B ). The walls of the sinusoids are composed of sinusoidal endothelial cells (SECs) that are separated by fenestrations of 50 to 150 nm in diameter. The fenestrations allow the passage of metabolites, plasma proteins, pharmaceutical molecules, lipoproteins, and other solutes into the space of Disse (SD) surrounding the sinusoids while retaining blood cells in the vessels. Larger macromolecules and potentially immunogenic peptides enter the SD by transcytosis through the SECs. Once in the SD, the molecules are taken up by hepatocytes.


Hepatocytes
Hepatocytes make up 75% to 80% of the total cellular volume of the liver. They are responsible for drug, protein, carbohydrate, lipid, and heme metabolism in addition to the synthesis of a variety of proteins necessary for the maintenance of homeostasis at baseline and in response to acute insults secondary to ischemia-reperfusion, viral and bacterial infections, and toxins. Hepatocytes are polarized with heterogenous plasma membranes to facilitate their varied functions. The basolateral (sinusoidal) portion of the membrane is in direct contact with the SD while the apical portion of the membrane makes up the bile canaliculus that drains bile to the ductules. Hepatocytes are divided into different zones based on their proximity to the portal triad. Zone 1 is periportal, zone 3 is around the central vein (perivenous or pericentral), and zone 2 is in between (midzone). Zone 3 hepatocytes are furthest away from the portal tracts and thus receive blood with a lower oxygen tension and nutrient content. The metabolic functions of the hepatocytes differ based on the zone in which they are located (see Fig. 16.3C ). This metabolic zonation increases the efficiency of carbohydrate, amino acid, lipid, and xenobiotic metabolism. Periportal (zone 1) hepatocytes are the major sites of aerobic metabolism, and process such as glycogen synthesis and sulfation whereas perivenous (zone 3) hepatocytes are the major sites of anaerobic metabolism, glycolysis, and glucuronidation. By virtue of their location, zone 3 hepatocytes are most sensitive to hypoxia.
Hepatic Stellate Cells
Hepatic stellate cells (HSCs) make up 8% to 10% of all resident liver cells. These specialized cells reside in the SD between liver sinusoidal endothelial cells (LSECs) and the hepatocytes. In the normal liver, HSCs are believed to be in a quiescent state. In the setting of liver injury, these cells become activated in response to cytokines and chemokines generated by hepatocytes, LSECs, as well as leukocytes and Kupffer cells. The stellate cells proliferate and differentiate into myofibroblasts participating in hepatic inflammation and fibrosis.
Myeloid Cells
The myeloid cells that can be found in the liver consist primarily of Kupffer cells (20%-30%) also known as resident tissue macrophages, in addition to dendritic cells and myeloid-derived suppressor cells. At first glance, it may seem that these cells have a less important role than hepatocytes and LSEC. However, while Kupffer cells constitute around 20% to 30% of nonparenchymal cells, they constitute 80% to 90% of all tissue macrophages. Kupffer cells reside in the portal and lobular liver sinusoids where they engulf both infectious and noninfectious particles by phagocytosis. Once phagocytosed, these particles are unable to induce proinflammatory responses in the liver. Thus by prevalence and location, these cells serve critical roles in innate and adaptive immunity by detoxification where they down-regulate potentially proinflammatory triggers that could disrupt hepatic homeostasis.
Dendritic cells and myeloid-derived suppressor cells are the least abundant of myeloid cells. Hepatic dendritic cells are present in the normal liver and reside in the portal area and are believed to promote tolerance to phagocytosed particles. Hepatic myeloid-derived supressor cells suppress immune response in the liver. In acute hepatitis they reduce inflammation and limit tissue injury. Their immune suppressive function has been associated with adverse effects in certain pathologic conditions. In chronic viral hepatitis, they may promote viral persistence. They have also been associated with suppression of immune response to hepatic tumors.
Lymphocytes
Cells of lymphatic origin that can be detected in the liver include natural killer (NK) cells, NK T cells (NKT), mucosal-associated invariant T cells, and γδ T cells in addition to major histocompatibility restricted CD4+ T cells, CD8+T cells, and B cells. These cells are distributed throughout the liver parenchyma and serve critical roles in the innate (NK, NKT, mucosal-associated invariant T cells, and γδ T cells) and adaptive (major histocompatibility restricted CD4+ T cells, CD8+T cells, and B cells) immune responses. These cells work primarily to maintain hepatic homeostasis by promoting tolerance to foreign substances. However, when necessary, these MHC-restricted cells can promote the clearance of foreign substances by expanding in response to them while recruiting additional cells from extrahepatic sources such as the lymph nodes and the spleen.
Hepatic Physiology
Drug Metabolism
The vast majority of drugs used in the conduct of anesthesia are metabolized in the liver. A variety of enzymes convert drug molecules into more water-soluble (hydrophilic) molecules or compounds to facilitate their excretion. These enzymes are designated as being either part of the Phase I pathway or Phase II pathway based on the types of reactions they mediate. Phase I enzymes consist of the cytochrome P450 family of enzymes that convert lipophilic drug molecules to hydrophilic molecules primarily through oxidation, reduction, or hydrolysis. Non-CYP450 enzymes include monoamine oxidases, alcohol dehydrogenases, and aldo-keto reductase. The phase II pathway consists of the conjugation of the products of the phase I pathway with hydrophilic endogenous moieties to make them more water-soluble. Polar molecules may undergo Phase II metabolism without having undergone Phase I metabolism. The most common Phase II reaction is glucuronidation, which is the conjugation of the drug compound to glucuronic acid. This reaction is carried out by a family of enzymes known as uridine 5′-diphospho-glucuronosyltransferases. Other Phase II enzymes include sulfotransferases (SULT), glutathione S-transferases (GST), and catechol O-methyltransferases. The phase III pathway involves the excretion of compounds into the sinusoids or canalicular bile by molecular transporters that are transmembrane proteins which facilitate the movement of large or ionized molecules across cell membranes. The majority of these transmembrane proteins are part of a superfamily of ATP-binding cassette (ABC) transporters that use ATP to actively transport molecules. Common ABC-transporters include multidrug resistance protein (MDR), cystic fibrosis transmembrane conductance regulator, and multidrug resistance–related protein (MRP).
Some orally administered medications undergo extensive metabolism in the gut or liver prior to entering the systemic circulation. This metabolism is termed the first-pass effect and is responsible for the lower oral bioavailability of these medications.
Drug metabolism is affected by a number of factors including genetic polymorphisms of metabolic enzymes, age, gender, pregnancy, liver disease, and concomitantly administered medications. The expression and function of Phase I and Phase II enzymes are reduced in neonates. The activities of some CYP450 enzymes are increased in women compared to men. Genetic polymorphisms in drug metabolizing enzymes and transporters can lead to wide variations in the pharmacokinetics of some drugs such as warfarin, with some patients having lower rates of metabolism based on the specific CYP450 polymorphism they carry. The concomitant administration of medications may also influence drug metabolism. A number of commonly encountered medications can serve as inducers or inhibitors of the enzymes involved in the different phases of drug metabolism. Table 16.1 lists some of the commonly used drugs, which are metabolized and excreted by each of the three phases along with drugs that may serve as inhibitors or inducers for each phase.
Enzymes | Substrates | Inhibitors | Inducers |
---|---|---|---|
Phase I | |||
CYP3A | Midazolam, buspirone, felodipine, lovastatin, eletriptan, sildenafil, simvastatin, triazolam | Ketoconazole, clarithromycin, itraconazole, saquinavir, fluconazole, grapefruit juice, tipranavir/ritonavir | Phenytoin, rifampin, St. John’s wort, efavirenz, etravirine, nafcillin, prednisone |
1A2 | Alosetron, caffeine, duloxetine, melatonin, ramelteon, tacrine, tizanidine | Ciprofloxacin, enoxacin, fluvoxamine, oral contraceptives, phenylpropanolamine, | Montelukast, phenytoin, smoking components of cigarettes |
2C8 | Repaglinide, paclitaxel | Gemfibrozil, fluvoxamine, ketoconazole, trimethoprim | Rifampin |
2C9 | Celecoxib, warfarin, phenytoin | Amiodarone, fluconazole, miconazole, oxandrolone, capecitabine, etravirine, fluvastatin, metronidazole, sulfinpyrazone, tigecycline | Carbamazepine, rifampin, aprepitant, bosentan, phenobarbital, St. John’s wort |
Phase II | |||
UGTs | Bilirubin, phenols, estradiols, opiates, and carboxylic acids | Paclitaxel, midazolam, cyclosporine A, ketoconazole, phenobarbital, and phenytoin | Bilirubin, phenobarbitone, rifampin |
SULTs | Phenols, alcohols, and amines | Flavonoids, mefenamic acids, salicylic acids, clomiphene, and danazol | Retinoic acid, methotrexate |
NATs | Para-aminobenzoic acid, para-aminosalicylic acids, para-aminoglutamate, sulfamethazine, isoniazid, hydralazine, and sulfonamides | Caffeic acid, esculetin, quercetin, genistein, scopoletin, and coumarin | Androgens, aminophylline |
GSTs | Epoxides, quinone, sulfoxides, esters, and peroxides | Phenols, quinone, vitamin C derivatives, dopamine, and trans retinoic acid | Extracts of broccoli, cabbage, Brussels sprouts, and grapefruit |
Phase III | |||
P-gp | Digoxin, loperamide, vinblastine, talinolol | Amiodarone, azithromycin, cyclosporine, diltiazem, dronedarone, erythromycin, itraconazole, ketoconazole, lopinavir/ritonavir, quinidine, verapamil | Avasimibe, carbamazepine, phenytoin, rifampin, St John’s wort, tipranavir/ritonavir |
See the pharmacokinetics chapter for further discussion of hepatic extraction ratio.
Protein Metabolism
The liver is responsible for the synthesis and catabolism of proteins, amino acids, and peptides. It is the site of synthesis for 80% to 90% of the circulating proteins including hormones, coagulant factors, cytokines, and chemokines. As such it plays a significant role in the functioning of the body. Albumin is the predominant protein produced by the liver, accounting for over 50% of total plasma protein. It functions to transport lipids and hormones and maintain blood volume. The liver plays a central role in protein degradation. Amino acids are catabolized through one of two reactions: deamination or transamination. Both reactions lead to the production of ammonia, which the liver converts to urea through the urea cycle. Urea is then excreted by the kidneys in the urine.
Carbohydrate Metabolism
The liver is primarily responsible for storing and releasing glucose to meet the body’s needs. In the postprandial state, the liver stores glucose through glycogenesis. Once the glycogen stores are complete, the liver converts excess glucose into fat through lipogenesis. In the fasting state, the liver provides the body with glucose by breaking down glycogen (glycogenolysis) or by generating glucose from carbohydrate precursors (gluconeogenesis).
Lipid Metabolism
The liver plays an important role in lipid metabolism. Nonesterified fatty acids can arise from the lipase-mediated breakdown of complex lipids, or from thioesterase-mediated hydrolysis of fatty acid-CoA. These fatty acids can enter the liver following oral intake or they can enter the liver following the breakdown of adipose tissue. In the liver, fatty acid oxidation is regulated by two main factors: the supply of fatty acids to the liver (via lipolysis), and the amount of microsomal esterification that occurs. Lipid metabolism is also influenced by the carbohydrate metabolism, as the acetyl-CoA formed during carbohydrate metabolism can be utilized to synthesize fatty acids. Fatty acids can undergo biotransformation to supply energy for the needs of the body. Alternatively, the liver can convert amino acids and intermediate products of carbohydrates into fats and transport them to the adipose tissues.
Bile and Enterohepatic Circulation
The adult liver produces approximately 400 to 600 mL of bile each day. Bile facilitates the excretion of toxins as well as the absorption of dietary fats. It is the mechanism of excretion for compounds with molecular weights greater than 300 to 500 Daltons that are not readily excreted by the kidneys. It is used to excrete a host of endogenous and exogenous compounds, including bile acids, bilirubin, phospholipids, cholesterol, drugs, toxins, steroid hormones, and water-insoluble porphyrins. Box 16.1 lists drugs, chemicals, and their metabolites that are excreted in the bile. The other major function of bile is to assist in the digestion and absorption of dietary fats, cholesterol, and vitamins. Bile is 95% water by volume, with the remainder consisting of bile acids, phospholipids, cholesterol, bilirubin, as well as other exogenous and endogenous substances. The two primary bile acids are cholic acid and chenodeoxycholic acid. Bile acids are synthesized by hepatocytes from cholesterol. They are then conjugated to reduce hepatotoxicity and increase solubility and secreted into the canaliculi. The canaliculi drain into the biliary ductules, which connect to form hepatic ducts. The walls of the intrahepatic bile ducts are made up of cholangiocytes that modify the volume and composition of the bile. The ducts ultimately form the left and right hepatic ducts, which join into the common hepatic duct. Bile is stored and concentrated in the gallbladder, which connects to the biliary tree through the cystic duct. The common hepatic duct and cystic duct join to form the CBD, which connects to the duodenum through the sphincter of Oddi (hepatopancreatic sphincter). Following the ingestion of food, fatty acids in the duodenum stimulate the release of cholecystokinin (CCK) which causes the gallbladder to contract and the sphincter of Oddi to relax leading to the release of bile into the duodenum. The bile acids emulsify dietary fats and facilitate their absorption. The vast majority (95%) of the bile acids released into the duodenum are reabsorbed in the terminal ileum and returned to the liver to be reused. This pathway for recycling bile acids is known as the enterohepatic circulation (EHC). Enterohepatic cycling can impact the pharmacokinetics and pharmacodynamics of drugs that undergo biliary excretion by increasing their bioavailability, reducing their elimination, as well as altering their plasma concentration curves. The effect of EHC on the properties of a drug depends on the physiologic activity of the excreted form of the drug (i.e., prodrug or activated form), the ease with which the excreted form is reabsorbed through the intestines, and whether it is recycled through the liver into the bile or the systemic circulation. In the case of some drugs, EHC can lead to secondary and tertiary peaks in plasma concentration as the drug is recycled into the system.
Amiodarone | Estrone | Phenol red |
Ampicillin | Ezetimibe | Phenolphthalein |
Benzylpenicillin | 2-Fluoro-β-alanine | Phenytoin |
Bilirubin | Gentamycin | Pivampicillin |
Bromocresol green | Glibenclamide (glyburide) | Rifamide |
Bromosulfophthalein | Gliclazide | Rifamycin |
Cefixime | Imipramine | Roquinimex |
Ceftriaxone | Indocyanine green | Rose bengal |
Cefatzidime | Indomethacin | Spironolactone |
Cephaloridine | Irinotecan | Sulfamethoxazole |
Cephamandole | Lanatoside C | Sulindac |
Cephazolin | Lorazepam | Sulbactam |
Chenodeoxycholic acid | Lomnetazepam | Temafloxacin |
Chloramphenicol | Methotrexate | Testosterone |
Chlortetracycline | Metronidazole | Tetracycline |
Clindamycin | Mezlocillin | Thiamphenicol |
Demethylchlortetracycline | Morphine | Tolfenamic acid |
Diazepam | Mycophenolic acid | Toremifene |
Digitoxin | Mycophenolate mofetil | Troglitazone |
Digoxin | Nortriptyline | Trovafloxacin |
Doxycycline | Novobiocin | Ursodeoxycholic acid |
Erythromycin | Oltiprazl | Valproic acid |
Estradiol | Pethidine (meperidine) | Warfarin |
Role of the Liver in Coagulation
The liver plays a significant role in the coagulation system. It synthesizes all coagulation factors except factors III (thromboplastin), IV (calcium), and VIII (von Willebrand factor [vWF]). It also synthesizes proteins that regulate coagulation and fibrinolysis such as protein S, protein C, plasminogen activator inhibitor, and antithrombin III. Furthermore, it removes activated clotting and fibrinolysis products through the hepatic reticuloendothelial system. A number of factors require vitamin K to become active. Coagulation factors II, VII, IX, X, as well as protein C and protein S undergo posttranslational modification with vitamin K to become active. Briefly, glutamic acid in the amino terminus of these proteins is converted to gamma-carboxyglutamic acid. These gamma-carboxylated procoagulants can then bind calcium ions and form bridges to phospholipid surfaces that are essential for the formation of activation complexes. Warfarin acts by inhibition gamma-carboxylation. In addition to these vitamin K–dependent factors, hepatocytes also synthesize factor V, XIII, fibrinogen, antithrombin, α 2 plasmin inhibitor, and plasminogen. Thrombomodulin, tissue plasminogen activator, tissue factor plasma inhibitor, vWF, and urokinase are not synthesized in the liver. Instead these proteins are synthesized in endothelial cells, whereas urokinase is expressed by endothelial cells, macrophages, and renal epithelial cells. Tissue plasminogen activator is primarily removed from the bloodstream through the hepatic reticuloendothelial system.
Heme Metabolism, Bilirubin, and Porphyrias
The liver is involved in both heme synthesis and metabolism. Eighty to 90% of heme synthesis takes place in the bone marrow with the resultant heme used to produce hemoglobin. Most of the remainder of the heme is produced in the liver and used primarily to synthesize cytochrome P450 enzymes. Whereas the rate of heme synthesis in the bone marrow is a function of the availability of iron, the rate of synthesis in the liver is a function of the available free heme pool in the body. Heme is synthesized through an eight-step enzymatic cascade known as the Shemin pathway. Synthesis begins with glycine and succinyl CoA and proceeds through porphyrinogen intermediaries. A deficiency in any of the enzymes involved in heme synthesis leads to the development of porphyria. The specific type of porphyria and its clinical manifestations depend on the specific enzyme that is deficient and the substrate that accumulates as a result. The most common porphyria is acute intermittent porphyria with an estimated incidence of 5 to 10 per 100,000. It is caused by a deficiency in porphobilinogen deaminase, which catalyzes the conversion of porphobilinogen to hydroxymethylbilane. Patients typically have adequate levels of the enzyme for heme homeostasis; however, in response to endogenous or exogenous triggers that induce the Shemin pathway, the capacity of the system is exceeded and they accumulate precursors leading to symptoms. Common triggers include erythromycin, trimethoprim, rifampicin, phenytoin, and barbiturates. Clinical symptoms of an attack include severe, poorly localized abdominal pain (in >90% of cases), nausea, vomiting, agitation, and confusion. Hyponatremia occurs in 40% of attacks. Change in urine color to dark red (especially on exposure to light) is a common finding. Treatment consists of discontinuing the triggering agent, administering pain medication, carbohydrates, and hematin.
Bilirubin is a product of heme catabolism. The primary source is senescent erythrocytes that are phagocytosed by macrophages in the spleen, liver, and bone marrow. The released heme is metabolized by heme oxygenase into bilirubin, yielding carbon monoxide and iron in the process. Unconjugated bilirubin is water insoluble and thus tightly bound to albumin in the circulation. Hepatocytes convert bilirubin into a water-soluble form by conjugating it to glucuronic acid via the enzyme glucuronyl transferase. Conjugated bilirubin is then transported across bile canaliculi and excreted in the bile. In the colon, bilirubin is deconjugated, metabolized by bacteria, and converted into urobilinogen. Urobilinogens are either reabsorbed through the EHC or excreted in the urine and stool, giving urine and stool their characteristic colors.
Hepatic Regulation of Hormones
The liver can participate in endocrine functions through hormone synthesis or hormone degradation. Hepatocytes synthesize hormones or prohormones such as hepcidin, insulin-like growth factor, and angiotensinogen, respectively. In addition to these hormones, thrombopoietin is also synthesized by hepatocytes and LSECs. These hormones and prohormones have specialized roles in the human body. Thus hepcidin is responsible for iron homeostasis and regulates intestinal iron absorption, plasma iron concentrations, and tissue iron distribution by inducing degradation of the hepcidin receptor, ferroportin. Insulin-like growth factor promotes systemic growth, especially bone growth in children. Angiotensinogen, the precursor of all angiotensin proteins, regulates the systemic blood pressure as well as the water and sodium composition of the body. Thrombopoietin regulates platelet production by stimulating production and differentiation of megakaryocytes. In addition to hormone synthesis, the liver participates in endocrine function by inactivating many hormones, including thyroxine, aldosterone, antidiuretic hormone, estrogens, androgens, and insulin.
Evaluation of the Liver
Clinical Assessment
There are often no signs or symptoms of liver disease until it is quite advanced. Even then, the only clues may be mild or nonspecific symptoms such as loss of appetite, fatigability, malaise, disrupted sleep patterns, or subtle cognitive changes. Major risk factors for liver disease include: alcohol use; illicit drug use; sexual promiscuity; blood transfusions; exposure to hepatotoxins; prior bouts of jaundice; and a family history of genetic diseases such as hemochromatosis, α 1 -antitrypsin deficiency, and Wilson disease. Patients with advanced liver disease may have these nonspecific symptoms, as well as pruritus, easy bruising, and changes in urine or stool color. In advanced liver disease physical examination findings include jaundice, scleral icterus, ascites, spider angiomas, xanthelasma, asterixis, and palmar erythema.
Standard Laboratory Tests
Standard panels used to evaluate the hepatobiliary system are often called “liver function tests” ( Table 16.2 ). In fact, these tests do not measure specific liver functions. Instead, they help define broad categories of hepatobiliary pathology: hepatitis, hepatobiliary dysfunction, or insufficient protein synthesis.
Blood Test | Predominant Abnormality | ||
---|---|---|---|
Bilirubin Overload (Hemolysis) | Hepatocellular Injury | Cholestasis | |
Aminotransferases | Normal | Increased: May be normal or decreased in advanced stages | Normal: May be increased in advanced stages |
Serum albumin | Normal | Decreased: May be normal in acute fulminant hepatic failure | Normal: May be decreased in advanced stages |
Prothrombin time ∗ | Normal | Prolonged | Normal: May be prolonged in advanced stages |
Bilirubin (main form present) | Unconjugated (also mild increase in conjugates) | Conjugated | Conjugated |
Alkaline phosphatase | Normal | Normal: May be increased by hepatic infiltrative disease | Increased |
γ-Glutamyl transpeptidase 5′-nucleotidase | Normal | Normal | Increased |
Blood urea nitrogen | Normal: May be increased by renal dysfunction | Normal: May be decreased by severe liver disease and normal kidney function | Normal |
BSP/ICG (dye) | Normal | Retention of dye | Normal or retention of dye |
∗ Used interchangeably with the international normalized ratio.
Detection of Hepatocellular Injury
Aminotransferases
Serum alanine aminotransferase (ALT) and aspartate aminotransferase (AST), formerly named serum glutamic-pyruvic transaminase and serum glutamic-oxaloacetic transaminase, respectively, are most commonly elevated because of hepatocellular injury. Both are aminotransferases, enzymes involved in gluconeogenesis. ALT is mainly a cytoplasmic liver enzyme. In contrast, cytoplasmic and mitochondrial isozymes of AST are found in many extrahepatic tissues, including the heart, skeletal muscle, brain, kidney, pancreas, adipose, and blood. Therefore isolated elevations of AST likely represent non-liver sources, but concomitant elevations in AST and ALT usually represent liver injury. Rarely, elevations in AST and ALT levels may result from muscle injury.
Practice guidelines provide recommendations for the evaluation of abnormal liver enzymes, based on the combination of clinical picture and degree of elevation of AST and ALT. Establishing normal reference ranges for AST and ALT have been complicated by studies with heterogeneous populations, since normal ranges differ with gender and body mass index (BMI). Nevertheless, multiple studies show that even mild elevations of AST and ALT above the upper limit of normal are associated with increased mortality. Some clinicians therefore argue that the normal limits should be lowered.
Elevated ALT and AST levels are sometimes described in qualitative terms, ranging from mild (>100 IU/L), to extreme (>2000 IU/L). The extent of aminotransferase elevation can sometimes aid in the differential diagnosis. Mild elevations in AST and ALT can arise from any hepatocyte injury. Large elevations often reflect acute hepatocyte ischemia. Extreme elevations signify massive hepatic necrosis, such as from fulminant viral hepatitis, severe drug-induced liver injury, or shock liver. However, aminotransferase levels do not reliably represent the extent of liver damage. Patients with so called “burnt out” livers, such as from chronic hepatitis, have insufficient functioning hepatocytes to bring about a transaminase increase.
The ratio of AST to ALT may help identify the cause of liver disease. Most causes of hepatic injury are associated with higher ALT than AST levels. However, Wilson disease and alcoholic liver disease are classically associated with an AST-ALT ratio greater than 1; in some cases, a ratio of 4 has been documented. Chronic hepatitis with minimal fibrosis has a low AST-ALT ratio; however when cirrhosis develops the ratio is greater than 1. Although the AST-ALT ratio may have insufficient predictive value as a sole marker, it can be used in combination with other noninvasive tests to predict the degree of fibrosis in patients with chronic hepatitis.
Lactate Dehydrogenase
Lactate dehydrogenase (LDH) is a nonspecific marker of hepatocellular injury. Extremely elevated LDH signifies massive hepatocyte damage, usually from ischemia or drug-induced hepatotoxicity (such as acetaminophen overdose). These patients will also have extreme elevations in AST and ALT. Elevated LDH concomitant with elevated alkaline phosphatase (AP) suggests malignant infiltration of the liver. Extrahepatic disorders that cause LDH elevation include hemolysis, rhabdomyolysis, tumor necrosis, renal infarction, acute stroke, and myocardial infarction.
Glutathione-S-Transferase
GST is a sensitive test for liver injury, with a half-life (60-90 minutes) much shorter than AST or ALT. Following hepatocyte damage, serum GST rises quickly and serial GST measurements can help monitor for disease recovery. The enzymes AST and ALT are found primarily in periportal hepatocytes (acinar zone 1), whereas GST is present in hepatocytes throughout the acinar zones. Since centrilobular/perivenous hepatocytes are most susceptible to hypoxic injury and acetaminophen toxicity, GST is a useful marker particularly in early stages of injury.
Detection of Cholestatic Disorders
Alkaline Phosphatase
AP isoenzymes are present in many tissues, including liver, bone, intestine, and placenta. In the liver, AP is present in the canalicular membranes of the hepatocytes. Mild elevations in AP may be normal, as AP varies with gender, age, blood type, and smoking status. Nonhepatic causes of AP elevation include bone disorders such as Paget disease, osteomalacia, and tumors of the bone; normal growth spurts in children; the third trimester of pregnancy; sepsis; renal failure; and some medications. Elevated AP from a hepatobiliary source is most commonly due to cholestatic disease and typically manifests as an increase to 2 to 4 times the upper limit of normal. Rarely, patients with cholestasis can have extreme elevations in AP (10 times the upper limit of normal). AP will also be elevated to a lesser extent in hepatocellular disease such as hepatitis. The ALT to AP ratio helps differentiate hepatocellular injury (ALT:AP > 5) from cholestatic disease (ALT:AP < 2) from a mixed picture.
AP elevation from cholestasis could be a result of either intrahepatic or extrahepatic biliary obstruction. Common causes include primary biliary cirrhosis, choledocholithiasis, and hepatic malignancy compressing small intrahepatic bile ducts. With a half-life of about 1 week, AP will be normal immediately after the onset of biliary obstruction, and it will remain elevated for days after resolution of obstruction.
The source of AP isoenzyme elevation can be determined by electrophoresis, but this test is expensive and not routinely available. More commonly clinicians confirm the hepatic origin of the AP elevation with other tests that indicate cholestatic disease. The enzymes 5′-nucleotidase and gamma-glutamyl transpeptidase are also elevated in cholestatic disorders, coincident with AP. Simultaneous elevation of these enzymes help identify liver as the source of AP elevation.
Serum Bilirubin
Serum bilirubin is the most commonly used test to monitor for excretory dysfunction of the liver. Bilirubin is measured in serum assays as either direct or indirect bilirubin. Direct bilirubin measures the water-soluble form, which interacts directly with the assay’s reagents. While direct bilirubin correlates with conjugated bilirubin levels, and indirect with unconjugated levels, the two terms are not synonymous. The level of unconjugated bilirubin is typically underestimated by the indirect bilirubin test. Distinguishing conjugated from unconjugated bilirubin is key to the differential diagnosis. The presence of bilirubin in the urine can also help differentiate the clinical cause. Bilirubinuria usually reflects conjugated hyperbilirubinemia, since only the water-soluble conjugated form is excreted by the kidney ( Fig.16.4 ).

Elevation in the concentration of unconjugated bilirubin is either due to excessive heme breakdown, or the inability of the liver to conjugate bilirubin. One major cause is hemolysis, in which increased erythrocyte breakdown creates more unconjugated bilirubin than the liver is able to conjugate. Massive hemolysis will cause elevations in both forms of bilirubin, but with unconjugated predominance. Gilbert syndrome is a benign condition with genetically low levels of the hepatic enzyme glucuronyl transferase, associated with mild or intermittent elevation in unconjugated bilirubin. Various drugs may also produce unconjugated hyperbilirubinemia. High levels of unconjugated bilirubin are neurotoxic, particularly in infants.
Conjugated hyperbilirubinemia occurs due to either problems with the secretion of conjugated bilirubin from hepatocytes into canalicular bile or to the blockage of bile flow within the hepatobiliary tree. This can be due to a genetic defect in the excretion of bilirubin, or cholestasis (either intrahepatic or extrahepatic). Intrahepatic cholestasis is caused by an inflammatory or infiltrative process compressing small intrahepatic bile ducts, while extrahepatic cholestasis is due to biliary obstruction such as from stones or a pancreatic mass. Primary sclerosing cholangitis (PSC) can involve both intra- and extrahepatic bile ducts.
Assessment of Hepatic Protein Synthesis
Serum Albumin
The serum albumin concentration is used to evaluate chronic liver disease and hepatocellular function (protein synthesis). However, it has poor specificity. Hypoalbuminemia has many other causes besides decreased protein synthesis, including increased catabolism, expansion of the plasma volume, renal losses, and maldistribution of total body albumin. The half-life of albumin in the serum is 20 days. As a result, changes in the synthetic function of the liver are not acutely reflected by the serum albumin concentration. Prealbumin is another protein synthesized in the liver that is also involved in transport and binding. It has a much shorter half-life than albumin. However, the level of prealbumin reflects the status of protein nutrition to a greater degree than liver synthetic function given its high percentage of essential amino acids.
Prothrombin Time
Hepatic synthetic function can also be assessed by measuring the levels of liver-derived coagulation factors. The liver synthesizes coagulation factors in great excess, thus the prothrombin time (PT) will remain normal until significant hepatic impairment occurs. Coagulation factors have shorter half-lives than albumin, ranging from 4 hours for factor VII to 4 days for fibrinogen. Thus when severe liver dysfunction occurs, PT (or the international normalized ratio [INR]) can reflect acute liver failure more quickly than can albumin. It is also used to monitor for restoration of hepatic function, often improving before other clinical signs of improvement occur. However, prolonged PT/INR is not specific for liver disease. It may also represent vitamin K deficiency, warfarin effect, or a genetic factor deficiency.
Testing for Diagnosis of Specific Diseases
In addition to the above standard laboratory tests, targeted testing can aid in the diagnosis of specific hepatobiliary diseases. Such testing includes serologic testing for viral and autoimmune diseases, genetic testing, and tumor marker assays. Viral markers, including antibodies, antigens, and genetic material, are used to diagnose hepatitis from hepatotropic viruses (A, B, C, D, and E) and herpesviruses such as cytomegalovirus and Epstein-Barr virus. Patients with acute or chronic hepatitis B infection and those with immunity due to resolved infection or vaccination can be distinguished based on measurements of hepatitis B surface antigens and surface and core antibodies ( Table 16.3 ). HBV DNA testing has also emerged to monitor treatment effect and the development of chronic hepatitis B after resolution of acute hepatitis.
Hepatitis B Virus Serologies | Interpretation |
---|---|
Hepatitis B surface antigen (HBsAg) | Acute or chronic infection |
Antibody to hepatitis B surface antigen (Anti-HBs) | Immunity due to recovery from infection or vaccination |
IgM antibody to hepatitis B core antigen (Anti-HBc IgM) | Previous or ongoing infection |
IgG antibody to hepatitis B core antigen (Anti-HBc IgG) | Acute infection |
Interpretation of Constellation of Results | |
HBsAg NEG Anti-HBs POS Anti-HBc IgG POS | Immune due to recovery |
HBsAg NEG Anti-HBs POS Anti-HBc IgG NEG | Immune by vaccination |
HBsAg POS Anti-HBs NEG Anti-HBc IgM POS | Acute hepatitis B infection |
HBsAg POS Anti-HBs NEG Anti-HBc IgG POS Anti-HBc IgM NEG | Chronic hepatitis B infection |
HBsAg NEG Anti-HBs NEG Anti-HBc IgG NEG | Not immune, potentially susceptible |
Testing for hepatitis C is recommended for those with various risk behaviors and exposures (such as history of intravenous drug use, long term hemodialysis, or HIV infection) and as part of an evaluation for unexplained liver disease. American guidelines also recommend one-time screening for hepatitis C in all persons in the United States born between 1945 and 1965, as this birth cohort has the highest prevalence of hepatitis C. Screening entails testing for antibodies to HCV; a positive screen indicates exposure to the virus. Active infection can then be confirmed with nucleic acid testing for HCV RNA. Sustained viral response to treatment is defined as the absence of HCV RNA detected at least 12 weeks after the completion of therapy. Currently, patients also receive HCV genotype testing prior to the initiation of treatment. New direct antiviral agents are highly effective in achieving sustained viral response for most genotypes and most clinical conditions. Therefore genotype testing may soon no longer be routinely required.
Rapid HCV antibody testing and point of care RNA tests are useful in community-based settings serving high-risk populations and remote settings without access to centralized laboratories. The measurement of HCV core antigen (HCVcAg) can diagnose active hepatitis C infection with high sensitivity and specificity as a single test, although it is currently not the standard of care. HCVcAg measurement is particularly useful as a rapid, single test for diagnosis in populations who are at high risk of loss to follow-up. However, it is not yet available as a point of care test.
Markers of hepatic malignancy include alpha fetoprotein (AFP) and protein induced by vitamin K absence or antagonist-II (PIVKA-II). AFP is a glycoprotein synthesized in the liver, fetal yolk sac, and GI tract. The normal value of AFP is less than 20 ng/mL. A significantly elevated concentration of AFP, often greater than 1000 ng/mL, is common in patients with hepatocellular carcinoma (HCC). AFP is also elevated in testicular germ cell tumors, and less frequently in prostate and other gastrointestinal cancers. The concentration of AFP is used to monitor HCC disease progression and response to treatment. It is also widely used to screen for HCC in high-risk patients, along with ultrasound. However, AFP’s role in HCC surveillance has recently been debated, as studies suggest that AFP has insufficient sensitivity as a screening tool for HCC, particularly for small and solitary tumors. AFP may be normal in 40% of HCC patients. PIVKA-II, also known as des-γ-carboxylated prothrombin, is a biomarker for HCC with high specificity. With a shorter half-life than AFP, PIVKA-II is useful in monitoring for treatment response and recurrence. PIVKA-II is also associated with worse survival. AFP and PIVKA-II combined can diagnose HCC with further increased sensitivity and specificity.
Testing in Management and Prognosis of Liver Disease
Laboratory tests can help define the type of liver dysfunction, and even identify specific causes. In addition, some of these markers are used to assess disease severity, monitor disease progression and treatment, and predict mortality. Taken alone, thrombocytopenia is the earliest sign of cirrhosis among the routine serum tests, indicating both decreased hepatic function and splenic sequestration from portal hypertension. Some markers are combined to create scoring systems, thereby improving the sensitivity and specificity of single tests. For example, the Model for End-Stage Liver Disease (MELD) score (a calculation that combines INR, bilirubin, and creatinine) was created to assess risk of mortality in cirrhotic patients undergoing transjugular intrahepatic portosystemic shunt (TIPS) procedures. The MELD score now is used for liver transplant listing, as it correlates with wait-list mortality. Standard tests such as INR, lactate and platelet count, as well as novel quantitative tests have been used to predict short-term outcome after liver transplantation. The HCC-MELD score, which combines AFP, MELD, and tumor size, can predict survival after liver transplantation in HCC patients. Elevated bilirubin predicts poor prognosis in acute or chronic liver disease, and AP and bilirubin together predict poor outcome in primary biliary cirrhosis.
Noninvasive Serum Testing for Fibrosis
Multiple models combining noninvasive measurements have been used to assess the severity of fibrosis. The goal of these models is to allow for disease staging (from mild fibrosis to cirrhosis) without requiring liver biopsy. Although liver biopsy remains the diagnostic gold standard, limitations include sampling error, subjectivity in interpretation, pain, bleeding, and cost. These models include various combinations of standard tests such as aminotransferases, platelet count, and INR; as well serum markers of extracellular matrix turnover such as alpha-2-macroglobulin, apolipoprotein A1, and hyaluronic acid. New commercial panels test an array of direct markers of collagen turnover, which correlate with fibrosis.
Quantitative Liver Tests
Quantitative liver function tests can estimate hepatocellular function by measuring the clearance of various substances metabolized by the liver. Two such substances are indocyanine green (ICG) and bromsulphalein. However, clearance techniques are imperfect tests, potentially influenced by extrahepatic uptake or clearance of the substance, changes in blood flow including portosystemic shunting, and other unknown factors. ICG is avidly extracted by the liver and undergoes minimal extrahepatic uptake and metabolism. Its elimination kinetics are expressed in the ICG plasma disappearance rate (PDR). It can be measured noninvasively by a transcutaneous method. The test is even sensitive to early changes in liver function, and it may be used to guide clinical management. By estimating functional hepatocellular mass, it may help predict outcomes after partial liver resections. It is also used as an early test of graft function following liver transplantation. However, like other highly extracted substances, ICG clearance is dependent on hepatic blood flow, and therefore reflects changes in both hepatic blood flow and hepatocyte function. In fact, ICG is also used specifically to test hepatic blood flow as discussed below. A drop in the ICG PDR may represent a reduction in hepatocellular function, a decrease in hepatic blood flow, or both. Bromsulphalein is another highly extracted substance that can be used to measure hepatic clearance. Bromsulphalein has been associated with severe systemic reactions, and therefore is largely no longer used.
The capacity of the liver to metabolize drugs can also be measured by caffeine clearance, galactose elimination, aminopyrine breath test, and monoethylglycinexylidide (MEGX). Caffeine clearance can be measured noninvasively through metabolites present in the saliva up to 24 hours after oral caffeine intake. MEGX is the primary metabolite of lidocaine and is measured in the serum after intravenous injection of lidocaine. MEGX concentration may be used to estimate liver function after partial hepatectomy and may have prognostic value in intensive care unit patients with liver dysfunction. MEGX was found to independently predict poor outcomes in patients with chronic hepatitis C, and it was more sensitive than standard liver tests in identifying these patients. Expensive and time consuming, quantitative tests currently remain primarily research tools and require further validation.
Measurement of Hepatic Blood Flow
Blood flow to the liver can be measured using clearance techniques, indicator dilution techniques, and direct measurements.
Clearance Techniques
Based upon the Fick principle, clearance techniques estimate the hepatic blood flow by measuring the rate of disappearance of substances that are exclusively and avidly cleared by the liver. Such substances with high extraction ratios include ICG, propranolol, lidocaine, and radiolabeled colloid particles. The primary limitation of clearance techniques is they assume normal hepatocyte function. Hepatic capacity to eliminate these substances can be variably or significantly diminished in liver disease. This is particularly problematic since liver dysfunction and altered hepatic flow are so often linked.
The dual cholate test measures the clearance of cholate, a bile salt, given in both oral and intravenous form. The oral cholate undergoes a high first-pass extraction and allows for a calculation of portal circulation. Clearance from the systemic circulation is measured using the intravenous cholate. One can quantify the degree of portal-systemic shunting and calculate a disease severity index. This index correlates with degree of fibrosis on liver biopsy and can predict risk of cirrhosis and poor clinical outcomes.
Indicator Dilution Techniques
Unlike clearance methods, indicator dilution techniques can measure hepatic blood flow even in the setting of liver dysfunction. A known quantity of a radiolabeled indicator (e.g., iodinated albumin) is injected into the portal system and hepatic artery. The concentration of that substance is then measured continuously from a hepatic vein. Hepatic blood flow can then be calculated by creating indicator dilution curves. The substance used should be resistant to hepatic clearance. Indicator dilution techniques also presume uniform liver perfusion, and therefore results could be altered in patients with shunting. These techniques are invasive and are still primarily research tools as well.
Direct Measurements
Blood flow through the portal vein or hepatic artery can be measured directly using electromagnetic or ultrasonic probes. These techniques are invasive and subject to significant error. The surgical procedures required to implant the probes can themselves alter hepatic blood flow. Once implanted, the probes are often left in place, and blood flow is then measured via telemetry. Implantable Doppler probes are sometimes used in patients immediately post-liver transplantation, and in patients who are at high risk for hepatic artery or portal vein thromboses.
Radiologic Methods
Radiologic techniques play important roles in diagnosing liver disease. Splenoportography evaluates the splenic and portal veins; it is useful in identifying varices and thromboses. Portal venography uses three-dimensional CT to create vascular maps of portosystemic collateral vessels. These noninvasive imaging techniques are particularly useful in surgical planning, such as before liver transplantation.
Inexpensive and routinely available, ultrasound can help diagnose cirrhosis with high sensitivity and specificity. Ultrasonic signs of cirrhosis include surface nodularity, hepatomegaly, and hypertrophy of the caudate lobe, as well as signs of portal hypertension, such as ascites, splenomegaly, and increased portal vein diameter. Doppler ultrasonography can show diminished portal flow and portal venous flow reversal. Fibrosis can also be estimated using elastography, a noninvasive measure of liver stiffness (LS). A shear wave is delivered across the liver via an ultrasound probe, and the wave propagation is then measured. Transient elastography is reproducible and easily performed in the office. Magnetic resonance elastography is especially useful in obese patients and those with ascites, where transient elastography is less accurate. Stiffness is a continuous measure which correlates with the histological stage of fibrosis, with high sensitivity and specificity. The combination of serum tests along with elastography offers much improved accuracy in diagnosing fibrosis. These tests are now widely used in practice, although they have not yet supplanted the role of liver biopsy.
Patients with chronic liver disease and chronic hepatitis B virus carriers are at increased risk for developing HCC and require regular screening for the cancer. Ultrasound is the most widely used screening tool for HCC worldwide. Multiphasic, contrast-enhanced CT and MRI are used to evaluate focal liver lesions suspicious for HCC with high sensitivity and specificity. While normal liver parenchyma receives only 25% blood flow from the hepatic artery (the rest via the portal vein), HCC cells receive their blood supply primarily from the hepatic artery. Contrast-enhanced imaging reflects this fact with HCCs showing hyperintensity in the arterial phase and washout during the portal venous phase. The Liver Imaging Reporting and Data System (LI-RADS) is a standardized tool for the radiologic classification of liver lesions in patients with chronic liver disease. Use of the LI-RADS has improved radiologic diagnostic accuracy and reduced the need for biopsy in these patients. In fact, confirmatory biopsy is rarely used, even prior to liver transplantation for HCC.
Hepatic Pathophysiology
Cholestatic Disease
Cholestasis is the impairment of bile production or flow. Cholestatic disorders are characterized primarily by elevations of serum AP and gamma-glutamyl transferase (GGT), with or without elevations in bilirubin. Cholestasis can present as an acute or chronic condition and is thought to affect up to 20% of the population. The impairment of flow leads to an increase in the concentration of bile salts in the serum and hepatocytes. As the bile salts accumulate in the liver, they dissolve hepatocyte cell membranes, leading to the release of AP and GGT, resulting in an increase in the serum concentrations of these enzymes. Serum concentrations of AST and ALT, which are found in the cytoplasm of hepatocytes, are not increased to as great an extent. As the disease progresses, the serum concentration of conjugated bilirubin increases, giving rise to jaundice. The majority of cases of cholestasis are benign; however, severe and prolonged cholestasis can lead to cirrhosis.
Signs and symptoms of cholestasis include fatigue, pruritus, dark urine, and pale stools. An elevation of AP on screening laboratory studies may be the first indication in asymptomatic patients. The evaluation consists of a history and physical exam followed by abdominal ultrasonography. Abdominal ultrasonography is used to distinguish between extrahepatic and intrahepatic cholestasis. Extrahepatic cholestasis results from mechanical obstruction of the extrahepatic bile ducts, typically by stones, strictures, or masses leading to ductal dilation. Definitive diagnosis and treatment is by endoscopic retrograde pancreatography (ERCP). If the etiology is unclear, further imaging can be conducted using endoscopic ultrasound or magnetic resonance cholangiopancreatography to identify the biliary lesion leading to obstruction. Normal imaging results indicate intrahepatic cholestasis. Intrahepatic cholestasis can have several etiologies including immune-mediated, infectious, drug-induced, paraneoplastic, and ischemic. The first step in the evaluation is the measurement of antimitochondrial antibody (AMA) titers to rule out primary biliary cholangitis (PBC). Further evaluation may require ERCP and liver biopsy.
Primary Biliary Cholangitis
Formerly known as primary biliary cirrhosis, PBC is an autoimmune disease that is characterized by the destruction of intrahepatic cholangiocytes and the presence of AMAs. It is one of the most common cholestatic liver disorders. Reported prevalence rates vary from 20 to 490 cases per million inhabitants with the highest rates reported in the United States. Over 90% of patients are female and the average age at diagnosis is typically in the sixth decade. The diagnosis is made based on an elevation of AP over 1.5 times the upper limit of normal for a duration greater than 24 weeks along with the presence of an AMA titer greater than 1:40. A liver biopsy in these patients will reveal interlobular bile duct lesions. Up to 60% of patients may be asymptomatic at diagnosis. If present, the most common symptoms at presentation are fatigue and pruritus. A large number of patients may present with other autoimmune disorders such as keratoconjunctivitis sicca and Raynaud phenomenon. PBC may be associated with osteopenia as well as hyperlipidemia. The disease develops as a result of the loss of tolerance to mitochondrial antigens, leading to autoimmune-mediated destruction of biliary epithelial cells (cholangiocytes). PBC can lead to cirrhosis and liver failure, ultimately requiring transplantation.
Treatment consists of ursodeoxycholic acid (UDCA) administration. UDCA, a bile acid, is thought to act by modifying the bile acid pool and reducing inflammation. Approximately 60% to 70% of patients with PBC respond to UDCA with improvement in serum AP and bilirubin levels and a delay in the progression to cirrhosis and liver transplantation. Patients who have a suboptimal response to UDCA have an increased risk of developing complications and liver failure. In these patients, the administration of obeticholic acid, a farnesoid-X receptor agonist, has been shown to reduce the serum levels of AP. Fibrates and corticosteroids have also shown benefit when combined with UDCA in patients who are unresponsive to UDCA alone.
Primary Sclerosing Cholangitis
PSC is a rare cholestatic, immune-mediated disease that occurs most frequently in men aged 30 to 40 years old. It is characterized by inflammation and fibrosis of the bile ducts. The majority of patients with PSC have ulcerative colitis as well. Approximately half of the patients are asymptomatic at diagnosis. The most common presenting symptoms are fatigue and pruritus. Blood tests demonstrate a cholestatic pattern. Patients may also have hypergammaglobulinemia and elevations in serum autoantibodies. Diagnosis is made based on the presence of multifocal strictures and segmental biliary dilations on cholangiography, and the exclusion of a number of other possible etiologies including bacterial cholangitis, choledocholithiasis, and surgical biliary trauma. Patients have an increased risk of developing hepatobiliary carcinoma. The disease is progressive, with biliary fibrosis leading to cirrhosis and ultimately end-stage liver disease (ESLD). Liver transplantation is the only effective therapy.
Cirrhosis
Cirrhosis is the common pathologic endpoint of a variety of mechanisms of hepatic injury. In the United States, it is the 14th most common cause of death and has a prevalence of 0.3%. The most common causes of cirrhosis in western countries are alcoholic liver disease, hepatitis C, and nonalcoholic steatohepatitis. In the Asia-Pacific region the most common cause is hepatitis B. The morbidity and mortality associated with cirrhosis result mainly from portal hypertension. Chronic liver disease promotes apoptosis and regeneration of hepatocytes. The resultant inflammation leads to parenchymal injury and fibrosis, causing distortion and obstruction of the hepatic vascular architecture. These structural changes increase hepatic resistance to portal blood flow (PBF) and give rise to portal hypertension. Hepatic vascular resistance is further increased due to hepatic endothelial dysfunction, which results in a reduction of vasodilator (primarily nitric oxide [NO]) production and an increase in vasoconstrictor (i.e., thromboxane) production. Portal hypertension and the release of angiogenic factors lead to the development of gastroesophageal varices as well as portosystemic shunts. As portal hypertension worsens, the locally released NO and prostaglandins cause the splanchnic circulation to vasodilate. This further increases portal inflow and exacerbates portal hypertension. Splanchnic vasodilation also leads to systemic hypotension that, in turn, increases sympathetic tone, activates the renin-angiotensin-aldosterone system, and increases antidiuretic hormone release, leading to the development of ascites and the hepatorenal syndrome (HRS). Other significant complications of cirrhosis and portal hypertension are spontaneous bacterial peritonitis, encephalopathy, HRS, portopulmonary hypertension (PoPH), cirrhotic cardiomyopathy, and HCC.
Laboratory abnormalities in cirrhotic patients include elevations in ALT, AST, AP, GGT, as well as serum bilirubin. Hypoalbuminemia and elevations in the PT are indications of hepatic synthetic dysfunction. Although liver biopsy is the gold standard for diagnosing cirrhosis, it is often not necessary in the presence of an appropriate patient history, and laboratory and radiologic data. In patients who are suspected of having cirrhosis, abdominal ultrasonography is used to aid in the diagnosis and evaluation for HCC and portal hypertension. Serologic studies and ultrasound-based techniques for evaluating hepatic fibrosis are also available.
The risk stratification of patients with cirrhosis is based on the presence and degree of portal hypertension, gastroesophageal varices, and synthetic dysfunction. There are two stages of cirrhosis: compensated and decompensated. Decompensated cirrhosis is characterized by the presence of ascites, variceal hemorrhage, and/or hepatic encephalopathy (HE). Compensated cirrhosis is characterized by the absence of these clinical conditions. While the median survival in patients with compensated cirrhosis is greater than 12 years, in those with decompensated cirrhosis it is approximately 2 years. Patients with compensated cirrhosis can be further stratified based on their degree of portal hypertension. Portal hypertension is defined as a hepatic venous pressure gradient (HVPG) greater than 5 mm Hg. Patients with an HVPG greater than 10 mm Hg are deemed to have clinically significant portal hypertension. They are at increased risk of developing varices, overt hepatic decompensation, postsurgical decompensation, and HCC compared to those with mild portal hypertension (HVPG between 5 mm Hg and 10 mm Hg). Although HVPG is the gold standard for monitoring portal hypertension, its invasive nature precludes its routine use. A noninvasive measure of clinically significant portal hypertension is LS using transient elastography, an ultrasound-based approach. An LS value greater than 20 kPa has been shown to effectively discriminate mild from clinically significant portal hypertension. Liver Doppler ultrasound may also aid in the diagnosis. The presence of portosystemic collaterals or reversal of portal venous flow on ultrasound imaging is also sufficient to diagnose clinically significant portal hypertension. In patients with clinically significant portal hypertension, the presence of gastroesophageal varices portends a worse prognosis.
The management of patients with compensated cirrhosis who don’t have gastroesophageal varices focuses on preventing the advancement of the disease by treating the underlying etiology. Patients with varices are treated with nonspecific beta-blockers (i.e., propranolol, timolol), carvedilol or endoscopic variceal ligation to prevent variceal hemorrhage. Nonspecific beta-blocker therapy reduces HVPG.
Acute variceal hemorrhage is a medical emergency. Management consists of airway protection, volume resuscitation, and endoscopy with variceal ligation. The concomitant initiation of antibiotic prophylaxis (ceftriaxone 1 g/24 h) and vasopressor infusion (somatostatin, octreotide, or terlipressin) has been shown to improve outcome and is the standard of care. Once stabilized, preemptive TIPS may be performed in patients who are at high risk of rebleeding.
Ascites
The presence of ascites is a marker of decompensated cirrhosis. The 1-year mortality rate of patients who develop ascites is 20%. Patients who develop ascites are at increased risk of developing further complications including spontaneous bacterial peritonitis and acute kidney injury (AKI). Management consists of dietary sodium restriction, nonspecific beta-blocker therapy to reduce portal pressure, and diuretic administration (spironolactone, furosemide). Midodrine can be added for refractory ascites. TIPS can improve transplant-free survival in certain patients with refractory ascites.
Renal Failure and Hepatorenal Syndrome
Up to 40% of patients admitted to the hospital with ESLD develop renal failure. Renal failure is a sign of advanced disease and has a poor prognosis in liver failure. Seventy to 80% of cases are precipitated by hypovolemia or bacterial infections. HRS is less common and associated with a worse prognosis. The diagnosis of HRS is one of exclusion. It is characterized by AKI in the absence of any apparent cause (shock, nephrotoxic drugs, obstruction, renal parenchymal disease) that is unresponsive to volume expansion and diuretic withdrawal. AKI is defined as a rise in creatinine greater than or equal to 0.3 mg/dL within 48 hours or a greater than 50% increase from baseline within 7 days. HRS is subcategorized into Type 1 and Type 2 based on the rapidity of onset and degree of injury. Type 1 is rapidly progressive with a doubling of serum creatinine within 2 weeks. It is usually associated with a precipitating factor and has a worse prognosis. The treatment for HRS is aimed at increasing perfusion pressure to the kidneys. For critically ill patients, norepinephrine infusion with albumin boluses can be used. In more stable patients, terlipressin (if available) or midodrine with octreotide is administered along with albumin. Dialysis may be used in the absence of response to medical therapy. Liver transplantation is the definitive treatment for HRS.
Hepatic Encephalopathy
HE is brain dysfunction secondary to hepatic insufficiency or portosystemic shunting. It is a sign of decompensated cirrhosis. It occurs in 30% to 40% of patients with cirrhosis and 50% of patients with portosystemic shunts. The clinical presentation is variable and can range from mild changes in personality to disorientation, somnolence, and coma. Asterixis is usually present in the early to middle stages. The severity of HE is typically graded from I to IV using the West Haven criteria with Grade I indicating mild symptoms and Grade IV, coma ( Table 16.4 ). Common precipitating factors of HE include infections, variceal hemorrhage, and diuretic overdose. Diagnosis is based on the exclusion of other causes of brain dysfunction and the application of clinical criteria. Treatment consists of amelioration of precipitating factors and the administration of lactulose, a nonabsorbable disaccharide. Rifaximin can be added on to prevent recurrence.
Grade | Description |
---|---|
I | Trivial lack of awareness; shortened attention span; disordered sleep |
II | Lethargy; behavioral change; asterixis |
III | Somnolence; confusion; gross disorientation; bizarre behavior |
IV | Coma |
Pulmonary Complications
Hepatopulmonary syndrome (HPS), PoPH, and hepatic hydrothorax (HH) are pulmonary conditions that can develop in patients with cirrhosis and advanced liver disease. HPS has been reported in up to 30% of patients presenting for liver transplantation evaluation and is associated with increased mortality independent of the severity of liver disease. It is defined as abnormal alveolar to arterial oxygen gradient (≥15 mm Hg) while breathing room air in sitting position. It is caused by intrapulmonary vascular dilation in the setting of cirrhosis. Its severity is determined by the PaO 2 : greater than 80 mm Hg is mild, 60 to 80 mm Hg is moderate, 50 to 60 mm Hg is severe, and less than 50 mm Hg is very severe. Patients may present with dyspnea at rest or on exertion. Approximately 25% of patients have platypnea (dyspnea when moving from supine to standing) or orthodeoxia (decrease in PaO 2 more than 5% or 4 mm Hg when moving from supine to standing). In advanced disease, patients may exhibit digital clubbing and cyanosis. Patients can be screened with pulse oximetry (SpO 2 < 96% on room air) and diagnosed based on PaO 2 values. Contrast-enhanced transthoracic echocardiography (TTE) may demonstrate intrapulmonary vascular dilation. No medical therapy has been shown to change outcome in patients with HPS. Supplemental oxygen is provided to maintain SpO 2 over 88%. Liver transplantation is the only effective treatment. As such, MELD exception points are granted for patients with severe HPS (PaO 2 50-60 mm Hg). Patients with very severe HPS (PaO 2 < 50 mm Hg) have an increased risk for complications and mortality following liver transplantation although this risk may be lower in experienced centers.
PoPH is pulmonary arterial hypertension in the setting of portal hypertension. It is found in 5% of patients presenting for liver transplant evaluation and, in the absence of treatment, is associated with a 1-year survival of 46%. The diagnosis is made by right heart catheterization showing a mean pulmonary artery pressure (mPAP) greater than 25 mm Hg, pulmonary vascular resistance (PVR) greater than 240 dynes/s/cm , and a pulmonary artery wedge pressure less than 15 mm Hg. PoPH is classified as mild (mPAP: 25-35 mm Hg), moderate (mPAP: 35-45 mm Hg), and severe (mPAP more than 45 mm Hg). The pathophysiology of the disease remains to be elucidated. Patients are typically screened using TTE to measure right ventricular systolic pressure (RVSP). Those with RVSP greater than 50 mm Hg undergo right heart catheterization to confirm the diagnosis and determine the degree of PoPH. PoPH is treated with phosphodiesterase-5 inhibitors, prostacyclin analogs, and endothelin receptor antagonists to reduce PVR. mPAP greater than 45 mm Hg is an absolute contraindication to liver transplantation. MELD exception is considered for treatment-responsive PoPH.
HH has a prevalence of 5% to 10% in cirrhotic patients. It develops due to the passage of ascitic fluid from the peritoneal cavity into the pleural space through defects in the diaphragm, most often on the right side. Patients may present with dyspnea, cough, chest discomfort, and hypoxia. Chest radiography and thoracentesis are used for diagnosis. Medical therapy consists of sodium restriction and diuretic administration. Refractory HH is treated with TIPS placement. For patients who have failed TIPS, VATS with pleurodesis is an option.
Hepatocellular Carcinoma
HCC is the most common primary liver malignancy and the third most common cause of cancer death globally. It occurs with greater frequency in men than woman and with a higher incidence in Asia and Africa, although the incidence has been increasing in the United States. Chronic liver disease is the most important risk factor for the development of HCC. Hepatitis B, hepatitis C, hemochromatosis, alcohol use, nonalcoholic fatty liver disease, diabetes mellitus, and obesity all increase the risk of developing HCC. Screening is recommended for all patients with cirrhosis at 6-month intervals using ultrasound with or without serum AFP measurement. Masses over 1 cm should be imaged with dynamic MRI or four-phase multidetector CT to make the diagnosis noninvasively. A percutaneous biopsy is obtained for nodules that have nontypical appearance on imaging. Staging is based on the size of the tumor, degree of extension into adjacent structures, presence of metastases, liver function, and the patient’s physical status. There is no universally accepted system for staging. The tumor, node, metastasis and the Barcelona staging system (BCLC) are two of the more commonly used systems.
Surgical resection of HCC is the definitive treatment for the disease. However, the diagnosis of HCC is typically made late in the course of the disease. In patients with preserved liver function and a solitary tumor confined to the liver, treatment with surgical resection carries a 5-year survival rate from 40% to 75%. Transplantation may be an option for patients with hepatic dysfunction and tumors confined to the liver that meet certain size criteria. Nonsurgical options for patients who are ineligible for resection or transplantation include radiofrequency ablation, transarterial chemoembolization, and systemic therapy.
Hepatic Effects of Anesthetic Agents
Inhalational Anesthetics
(See also chapter on pharmacokinetics/dynamics of volatile anesthetics.)
The hepatic effects of volatile anesthetics result primarily from alterations in liver perfusion. Total hepatic blood flow (THBF) is the sum of PBF and hepatic arterial blood flow (HABF). Reductions in PBF are matched by increases in HABF to maintain THBF through the hepatic arterial buffer response (HABR). Volatile anesthetics reduce mean arterial pressure (MAP) and CO, leading to a reduction in PBF in a dose-dependent manner. The HABR is preserved with isoflurane, sevoflurane, and desflurane leading to preservation of THBF, but not with halothane.
Xenon is an inert gas that can been used as an anesthetic. It has favorable anesthetic properties including a low blood-gas partition coefficient (0.115) and a stable hemodynamic profile. Hepatic perfusion during xenon anesthesia has been studied in pigs. In one study using radiolabeled microspheres, there was no difference in hepatic perfusion in pigs anesthetized with xenon when compared to propofol. However, a second study using ICG reported a 17% reduction in portal venous blood flow in pigs anesthetized with a ketamine infusion that received a xenon anesthetic compared to controls. They reported no significant difference in hepatic arterial perfusion or hepatic function. In a porcine model, the use of xenon anesthesia was associated with significantly higher hepatic venous oxygen content when compared with propofol. It is not clear that there are relevant clinical consequences to these findings. The successful use of xenon anesthesia for the conduct of liver transplantation has been reported in a series of four cases.
In general, intravenous anesthetics such as propofol, thiopental, etomidate, and methohexital do not adversely affect the liver. Furthermore, the pharmacokinetic properties of these agents are unchanged in patients with cirrhosis. Cirrhotic patients may be more sensitive to their central nervous system depressant effects. Propofol has been found to increase hepatic blood flow and oxygen consumption in both animal and human studies that have measured flow using radiolabeled microspheres, Doppler, and electromagnetic flow meters. Studies using ICG clearance to measure hepatic flow, however, have reported that propofol reduced hepatic flow. These findings were confounded by the fact that propofol itself interferes with ICG clearance. Clinically, propofol has no adverse effect on hepatic function. Midazolam elimination is impaired in liver dysfunction leading to prolongation of sedative effects. Dexmedetomidine elimination is reduced in patients with hepatic impairment, necessitating dose reduction.
In patients with significant cirrhosis, the metabolism of morphine, meperidine, and alfentanil is reduced and their durations of action are prolonged. In patients with mild hepatic insufficiency, the pharmacokinetics of fentanyl, sufentanil, and remifentanil are unchanged when compared to normal controls.
Reduced levels of pseudocholinesterase in patients with hepatic dysfunction may prolong the effects of succinylcholine and mivacurium. The time to recovery from cisatracurium is unchanged as it is subject to Hoffman degradation.
Aminosteroid neuromuscular blocking agents (vecuronium, rocuronium, pancuronium) have a larger volume of distribution in cirrhotic patients. They have a slower rate of onset and a longer duration of action, necessitating judicious dosing and close train-of-four monitoring. Sugammadex has been shown to be effective for reversing rocuronium in patients with hepatic dysfunction. The use of neostigmine for reversal is likewise unaffected by the presence of hepatic dysfunction.
Five studies have examined the effect of epidural anesthesia on hepatic blood flow. Four have shown a reduction in hepatic blood flow in response to lumbar or thoracic epidural anesthesia when measured using the PDR of ICG and transgastric hepatic vein Doppler. In one study, blood flow was restored with the infusion of colloid and dopamine. Maintenance of MAP with ephedrine or norepinephrine further reduced hepatic blood flow. Unlike these studies, a study by Kortgen et al. found that thoracic epidural anesthesia increased hepatic blood flow when measured by PDR-ICG, whereas lumbar epidural anesthesia resulted in an overall reduction. The clinical significance of these findings is uncertain.
Nonhepatic Surgery in Patients With Liver Disease
The preoperative evaluation of patients with liver disease should determine the severity of the disease and the presence of comorbid conditions associated with hepatic dysfunction. Given the mortality risk, elective surgery is contraindicated in patients with acute hepatitis or acute liver failure. In the absence of cirrhosis and significant hepatic dysfunction, patients with chronic liver disease generally have no increased risk for undergoing elective surgery. Patients with cirrhosis who undergo nonhepatic surgery have a higher incidence of postoperative morbidity and mortality when compared to patients without cirrhosis. A wide range of mortality rates has been reported in the literature (1%-50%), mostly in small, uncontrolled, institutional studies spanning 30 years. Mortality in these patients is a function of the severity of liver disease, its attendant comorbidities, and the type of surgical procedure. The largest study of in-hospital mortality in patients with cirrhosis used data from the Nationwide Inpatient Sample. The outcomes of 22,569 cirrhotic patients undergoing one of four index operations (cholecystectomy, colectomy, coronary artery bypass grafting [CABG], and abdominal aortic aneurysm repair) from 1998 to 2005 were compared to those of 2.8 million control patients. It was found that 4214 of the cirrhotic patients had portal hypertension. Patients with cirrhosis had a three- to eight-fold increase in the risk of in-hospital mortality, depending on the operation, compared to control patients. Mortality risk worsened in those with more severe liver disease, ranging from 12- to 23-fold, depending on the specific operation. The greatest risk was seen in patients undergoing CABG. A Taiwanese study comparing 24,282 cirrhotic patients undergoing major nonhepatic surgery with 97,128 matched control patients found an over two-fold increased risk of 30-day mortality in patients with cirrhosis (1.2% vs. 0.7%). Mortality was further increased in those cirrhotic patients with viral hepatitis, jaundice, ascites, gastrointestinal hemorrhage, and hepatic coma.
In cirrhotic patients undergoing nonhepatic surgery, the Child-Turcotte-Pugh (CTP) and MELD scores have been shown to identify those who are at higher risk of morbidity and mortality. The CTP score is calculated based on the values of five variables: degree of HE, degree of ascites, INR, serum albumin, and total bilirubin ( Table 16.5 ). The score is then used to classify the patient into one of three groups: Class A (score of 5-6), Class B (score of 7-9), and Class C (score > 10). A higher CTP class is associated with a greater risk of mortality. The mortality risk associated with each CTP class has decreased in more recent studies. In early retrospective series of cirrhotic patients undergoing abdominal surgery, the mortality risks associated with CTP Class A, B, and C were 10%, 30%, and 73% to 82%, respectively. In a more recent series of 194 patients undergoing 212 general surgical procedures, 30-day mortality was reported to be 6%, 13%, and 53% for CTP Classes A, B, and C, respectively. The risk has been found to be even lower in patients undergoing laparoscopic procedures (Class A: 2%; Class B: 12%; Class C: 12%), suggesting that improvements in perioperative care and surgical techniques have made surgery safer for patients with cirrhosis. A disadvantage of the CTP score is that the grading of encephalopathy and ascites is subjective. Furthermore, the score doesn’t take into account other predictors of operative risk such as the type of surgery or the etiology of the cirrhosis.
Points assigned | |||
---|---|---|---|
1 | 2 | 3 | |
Ascites | Absent | Slight | Moderate |
Bilirubin | <2 mg/dL | 2-3 mg/dL | >3 mg/dL |
Albumin | >3.5 g/dL | 2.8-3.5 g/dL | <2.8 g/dL |
prothrombin Time | |||
Seconds over control | <4 | 4-6 | >6 |
International normalized ratio | <1.7 | 1.7-2.3 | >2.3 |
Encephalopathy | None | Grades 1-2 | Grades 3-4 |
Point total | CTP class | Operative mortality | |
5-6 | A | 2-10% | |
7-9 | B | 12-31% | |
10-15 | C | 12-82% |
As described above, the MELD score, calculated using the patient’s INR, serum creatinine, serum bilirubin, and etiology of liver disease ( Fig. 16.5 ) is a validated measure of mortality risk in patients with ESLD and is the basis of the liver allocation system for transplantation. A number of studies have validated the MELD score as a predictor of risk in cirrhotic patients undergoing abdominal, orthopedic, and cardiac surgery. In a large, retrospective study of cirrhotic patients undergoing major abdominal, orthopedic, or cardiac surgery, MELD score was a predictor for mortality at 30 days, 90 days, 1 year, and 5 years. The 30-day mortality ranged from 5.8% in patients with a MELD under 8 to over 50% in patients with a MELD score greater than 20. The incorporation of serum sodium into the MELD score (MELD-Na) as well as a combination of serum sodium and patient age (integrated MELD or iMELD) have been shown to improve the accuracy of predictions of survival over MELD. The use of MELD-Na and iMELD may allow more accurate prediction of mortality following nonhepatic surgery; however more studies are needed.

Studies comparing the predictive power of CTP to MELD have yielded conflicting results likely due to small sample sizes and differences in primary outcome measures and surgical procedures. Small studies in cardiac and general surgical patients have found the abilities of CTP and MELD to predict postoperative mortality to be comparable. A study in cirrhotic patients undergoing extrahepatic abdominal surgery found MELD to be a better predictor than CTP for mortality or hepatic decompensation within 90 days of surgery. A more recent study found neither to be a good predictor for death and hepatic decompensation in cirrhotic patients undergoing elective surgery requiring general anesthesia. It has been suggested that both scores should be used in conjunction with other available patient data when attempting to risk-stratify cirrhotic patients for nonhepatic surgery.
Apart from the severity of cirrhosis and the specific surgical procedure, risk factors associated with increased postoperative morbidity and mortality in cirrhotic patients include emergency surgery, American Society of Anesthesiologists (ASA) physical status classification, the presence of renal insufficiency, male gender, and advanced age.
In addition to determining the severity of cirrhosis, the preoperative evaluation should determine the presence and severity of comorbidities associated with cirrhosis, including HE, pulmonary disease, cardiac disease, and renal dysfunction (see previous discussion). Preoperative laboratory evaluation should include a complete blood count to evaluate for anemia, thrombocytopenia, and leukocytosis. Additionally, INR, fibrinogen, serum electrolytes, creatinine, glucose, transaminases, bilirubin, and albumin should be measured.
Anesthetic Considerations for Procedures Involving the Liver
Transjugular Intrahepatic Portosystemic Shunt
TIPS placement is a catheter-based, endovascular procedure during which a shunt is created between the portal and hepatic venous systems through the parenchyma of the liver ( Fig. 16.6 ). The goal of the procedure is to reduce portal hypertension. The main indications for TIPS are the secondary prevention of variceal hemorrhage and the management of refractory ascites. During acute variceal hemorrhage in high-risk patients (HVPG > 20), TIPS placement following endoscopic intervention has been shown to be reduce rebleeding. Contraindications to the procedure include congestive heart failure, tricuspid regurgitation, and moderate to severe pulmonary hypertension. During the procedure, a catheter is typically inserted through the right internal jugular vein into a hepatic venous branch. A needle is then passed through the hepatic vein into the portal vein. The tract is dilated and a stent is placed across it. HVPG should be less than 12 mm Hg following the procedure. The incidence of major complications such as intra abdominal hemorrhage is on the order of 1% to 2%.

TIPS may be carried out electively or emergently. The preoperative assessment should determine the extent of liver dysfunction and associated morbidity. Patients with advanced liver disease may be in a hyperdynamic state. They may have significant ascites with reductions in FRC as well as HH or HPS. Pulmonary function may be further compromised when the patient is in a supine position for the procedure. Patients may also have renal dysfunction, anemia, coagulopathy, and thrombocytopenia. Laboratory studies should screen for anemia, thrombocytopenia, and coagulopathy, as well as hyponatremia and elevations of creatinine and potassium. Patients should be transfused for a hemoglobin concentration less than 7 to 9 mg/dL, INR greater than 2, and a platelet count less than 50,000/μL. The procedure can be conducted under local anesthesia, sedation, or general anesthesia. Consideration should be given to the acuity of the patient, their ability to tolerate supine positioning, and the anticipated length of the case. In patients with significant ascites or recent variceal hemorrhage, general anesthesia with rapid sequence induction for airway protection is preferred. Intraoperative pain may be experienced during the establishment of the intrahepatic shunt and the dilation of the stent. In a series of 150 patients undergoing TIPS with general anesthesia using TIVA, the authors reported a postoperative ICU admission rate of 6.6% primarily for intraoperative hemodynamic instability. They advocated for the use of general anesthesia for all TIPS procedures, given the concerns for airway protection, ability to maintain supine position, and pain associated with the procedure. Postprocedure complications include heart failure due to increased venous return, HE, contrast-induced nephropathy, hemolytic anemia, and sepsis.
Hepatic Resection
The most common indications for hepatic resection are for the treatment of secondary metastases (i.e., metastatic colon cancer), primary hepatic malignancies, biliary tract malignancies, and benign hepatic masses. Hepatic resection is carried out along the functional segments of the liver. The liver is divided into eight functional segments based on the distribution of blood supply and biliary drainage. The left liver is comprised of segments II, III, and IV, while the right liver is comprised of segments V, VI, VII, and VIII. Segment I is the caudate lobe (see Fig.16.1 ). Right hepatectomy involves resection of segments V-VIII. Left hepatectomy involves resection of segments II-IV. Right lobectomy, also described as an extended right hepatectomy or right trisegmentectomy, involves resection of segments IV-VIII with or without segment I. An extended left hepatectomy (left trisegmentectomy) is the resection of segments II-V and VIII. Mortality and morbidity rates are impacted by the extent of the resection. A review of the 4881 hepatic resections over a 5-year period in the American College of Surgeons – National Surgical Quality Improvement Program database reported 30-day mortality and morbidity rates of 1.9% and 13.1%, respectively, for patients undergoing partial hepatectomy. Those mortality and morbidity rates increased significantly for patients undergoing extended hepatectomies (lobectomies or trisegmentectomies) to 5.8% and 22.5%, respectively. Other factors which have been shown to impact outcomes are the case volume of the performing center, patient age, history of cardiac, pulmonary or renal disease, blood loss, the presence of ascites, and reduced hepatic function.
Laparoscopic hepatic resection is considered most appropriate for small (<5 cm), solitary lesions located in peripheral segments (II-VI), when performed in experienced institutions. More extensive resections have been reported in experienced centers. A metaanalysis of 83 comparative case series (2900 patients) found a significantly lower rate of complications, transfusions, blood loss, and hospital stay in case-matched cohort of patients undergoing laparoscopic liver resection compared to open liver resection. To date, the only randomized, controlled trial comparing laparoscopic to open liver resection found a significant reduction in postoperative complications in patients undergoing laparoscopic hepatic resection (19% vs. 31%; 95% confidence interval [CI], 1.67-21.8; P = .021) for colorectal cancer liver metastases requiring resection of fewer than three consecutive segments. Although the hospital length of stay was significantly shorter, there were no differences in blood loss or mortality. Conversion from a laparoscopic to an open procedure is usually due to intraoperative hemorrhage and is reported to occur in 6% to 14% of cases. Risk factors for conversion include elevated BMI, increased age, diabetes mellitus, hypertension, and large tumor size. A number of recent observational, retrospective studies have described the performance of robot-assisted hepatectomy for both minor and major resections. A metaanalysis of seven studies published between 2010 and 2014, comparing laparoscopic to robotic hepatectomy, found that the laparoscopic approach is associated with significant reductions in blood loss and operative time without any difference in the conversion rate, postoperative morbidity, and the hospital length of stay.
The preoperative evaluation of the patient should focus on determining the severity of liver disease and the presence of other comorbidities. Both cirrhosis and steatosis have been associated with increased mortality in patients undergoing liver resection. The decision to proceed is made based on the anticipated future liver remnant (FLR) that will remain following resection. In patients with an anticipated FLR size that is considered unsafe, selective portal vein embolization (PVE) may be conducted in the weeks prior to the hepatic resection. Disruption of portal flow to the diseased portion of the liver leads to atrophy of the portion to be resected and hypertrophy of the FLR, allowing the patient to have adequate liver mass following resection. PVE is typically indicated for patients with an anticipated FLR less than 20% in the absence of cirrhosis, and less than 40% in the presence of cirrhosis. Further preoperative evaluation and risk assessment should take place as outlined above. Laboratory investigations prior to hepatic resection should include a complete blood count, serum electrolytes, liver chemistries, albumin, coagulation studies, and a type and screen.
Strategies for Minimizing Blood Loss
Intraoperative blood loss during liver resection is associated with an increased risk of morbidity and mortality. Several preoperative factors have been associated with an increased risk of transfusion. These include preoperative anemia, the need for an extrahepatic procedure, the need for caval exposure, major hepatectomy (>3 segments), tumor size, thrombocytopenia, cirrhosis, and a repeat operation. For patients at increased risk of significant intraoperative hemorrhage, the use of techniques such as acute normovolemic hemodilution and intraoperative cell salvage have been shown to reduce the transfusion of allogeneic units.
A number of strategies have been adopted to reduce blood loss in open hepatic resection and case series from high-volume centers routinely report losses less than 500 mL. Such strategies include the use of temporary hepatic vascular occlusion and the intraoperative maintenance of low central venous pressure (CVP).
Hepatic vascular occlusion techniques may be used by surgeons to limit blood loss by limiting blood flow to the liver. Although not routinely used in simple resections, familiarity with these techniques is important as they may be employed in more complex resections. The most commonly used vascular occlusion technique is occlusion of the hepatic artery and portal vein by clamping the hepatoduodenal ligament. Otherwise known as the Pringle maneuver, total hepatic inflow occlusion was first described in 1908 as a means of controlling hemorrhage in hepatic trauma ( Fig.16.7A ). The drawback of this maneuver is the potential for hepatic ischemia and reperfusion injury. To mitigate this effect, surgeons typically make use of intermittent clamping with 15- to 20-minute intervals of clamping interspersed with 5- to 10-minute intervals of unclamping for a total ischemic time of less than 120 minutes. Tolerance of longer cumulative ischemic times has been reported. The application of sevoflurane for 30 minutes of preconditioning prior to the Pringle maneuver has been shown in one single-center randomized, controlled trial to significantly reduce postoperative transaminitis and complications in cases with greater than 30 minutes of continuous inflow occlusion. In a subsequent three-armed randomized controlled trial, the same group reported reductions in postoperative transaminitis and complications when sevoflurane was administered after the Pringle maneuver (“post-conditioning”) or with the use of intermittent clamping.

The utility of the Pringle maneuver for reducing blood loss in modern hepatic resection has been called into question. While two early randomized, controlled trials showed a significant reduction in blood loss with intermittent Pringle maneuver compared to no inflow occlusion, three, more recent, randomized controlled trials have reported no benefit. The median blood loss values reported in these three more recent trials were much lower than those reported in the initial two studies. This finding suggests that in the context of current intraoperative approaches to hepatic resection, the routine use of the Pringle maneuver may not be necessary.
An alternative technique that may be utilized is hemi-hepatic inflow occlusion. This maneuver involves exclusively clamping the branches of the portal vein and hepatic artery which supply the hemi-liver which is being resected in order to reduce ischemic injury to the remnant liver (see Fig. 16.7B and C ). Hemi-hepatic inflow occlusion hasn’t been shown to reduce blood loss when compared to Pringle maneuver. Total hepatic vascular exclusion (THVE) involves occlusion of the suprahepatic and infrahepatic IVC in addition to the portal vein and hepatic artery (see Fig. 16.7D ) THVE can result in significant hypotension due to the reduction in venous return as well as increased morbidity. It has not been shown to offer any benefit compared to the Pringle maneuver and its use is typically reserved for patients with tumor extension into the IVC. In such complex resections, it has been combined with hypothermic portal perfusion and venovenous bypass to mitigate the risks of hypotension and hepatic ischemia; however the reported mortality is high. To avoid the hemodynamic effects of THVE, selective hepatic vascular exclusion (SHVE), which involves clamping the hepatic veins instead of the IVC, may be employed by the surgeon. Some studies have shown that SHVE results in fewer complications than THVE; however it is technically demanding and has a limited role in uncomplicated hepatic resections.
Low Central Venous Pressure
While the Pringle maneuver occludes inflow to the liver, retrograde flow through the hepatic veins can still lead to substantial venous hemorrhage. The pressure in the hepatic veins is directly related to vena caval pressure, hence the strategy of maintaining a low CVP (<5 mm Hg) to minimize intraoperative blood loss. Retrospective observational studies have reported reduced blood loss in patients managed with CVP less than 5 mm Hg without any significant adverse events. Two randomized controlled trials comparing patients with CVP under 5 mm Hg with liberal volume administration have shown reductions in both intraoperative blood loss and the need for transfusion. These studies have all been at risk for significant bias. Furthermore, in some of the trials the difference in estimated blood loss, though statistically significant, was clinically inconsequential. Although standard practice in hepatic resections is to maintain low CVP, some studies have found no association between CVP and intraoperative blood loss. In retrospective studies of patients undergoing donor hepatectomies, no correlation was found between the CVP and intraoperative blood loss. This finding may be due to the fact that living liver donors are healthy patients with normal livers. The largest of these studies consisted of over 900 patients. Although it found no correlation between CVP and estimated blood loss, nearly all of the patients were managed with CVPs less than 10 mm Hg, and the majority had CVPs in the 4 to 6 mm Hg range.
A number of techniques have been described for reducing CVP during hepatic resection. The most commonly reported approach is the use of intraoperative fluid restriction with rates of 1 mL/kg/h. Vasopressors are used as needed to maintain SBP over 90 mm Hg and urine output more than 25 mL/h. Though seldom required, vasodilation using nitroglycerin or morphine, or forced diuresis with furosemide, can be instituted if fluid restriction is insufficient. The use of milrinone, reverse Trendelenburg position, and epidural anesthesia have also been described, though there is insufficient data to recommend one particular approach.
Clamping of the infrahepatic IVC (IIVC) has been described as a means of minimizing hemorrhage in the setting of an elevated CVP despite the interventions described earlier (see Fig. 16.7E ). A number of randomized controlled trials have demonstrated a significant reduction in blood loss and transfusion with the use of IIVC clamping in patients who tolerate the clamp or have a CVP greater than 5 mm Hg. A metaanalysis of these trials, comprising of 714 patients, showed a significant reduction in intraoperative blood loss (mean difference −353 mL) with no difference in the rates of postoperative complications. Of note, in pooled analysis, there was no significant difference in reported CVP values between the two groups.
Other Approaches to Reduce Blood Loss
Two multicenter, randomized, placebo-controlled trials have examined the efficacy of prophylactic recombinant Factor VIIa (rFVIIa) administration to reduce transfusion patients undergoing hepatic resection. The studies found no benefit of prophylactic rFVIIa with respect to mortality, red blood cell transfusions, or adverse events. A single randomized, controlled trial in 214 patients undergoing primarily minor hepatic resections (82% of cases) found that the administration of tranexamic acid (TXA) resulted in fewer transfusions and less blood loss compared to placebo. In the study group, 500 mg of TXA was administered intravenously prior to incision followed by 250 mg every 6 hours for 3 days.
A recent Cochrane database analysis of 67 randomized clinical trials of methods to decrease blood loss during liver resection found a high risk of bias in all trials. Based on the outcomes that were available in more than one trial, there is low-quality evidence that the “clamp and crush” parenchymal resection technique may be associated with fewer adverse events than radiofrequency resection techniques. They reported very low quality evidence that blood loss, operating time, and total hospital stay were reduced with intraoperative low CVP management.
Anesthetic Management
The anesthetic management of these cases should be tailored to the comorbidities of the patients, the surgical approach (open, laparoscopic), the anticipated extent of resection, and the need for vascular occlusive maneuvers. Adequate venous access should be obtained for the anticipated degree of hemorrhage. In cases where significant blood loss is anticipated, red blood cell salvage can be used and consideration should be given to the use of acute normovolemic hemodilution. In addition to standard ASA monitors, intraarterial blood pressure monitoring should be used in cases during which significant hemorrhage or the need for vascular occlusion maneuvers is anticipated. Central venous catheters may be inserted for both vascular access and CVP monitoring, although some high-volume centers forgo the routine use of CVP monitoring. Peripheral venous pressure monitoring may be considered as an alternative. Values are obtained through transduction of an antecubital vein and have been shown to correlate with the CVP in patients undergoing hepatic resection. Stroke volume variation (SVV) has been investigated as an alternative to CVP for volume management in patients undergoing liver resection. A study of patients undergoing donor hepatectomy showed an SVV value ≤6% to be predictive of blood loss over 700 mL. In two studies, targeting SVV values greater than 12% to 15% in patients undergoing open or laparoscopic hepatic resection yielded blood loss comparable to, or better than, maintaining low CVP. In a study using milrinone to reduce CVP, SVV of 9% yielded a favorable surgical field. No correlation with CVP was noted in this study, possibly due to the lusitropic effects of milrinone. The use of transesophageal echocardiography may be considered in patients with cardiac risk factors, although care must be taken in the presence of significant esophageal varices.
Hepatic resection may be associated with hemorrhage and, in extensive cases, the development of coagulopathy. There is insufficient data to recommend the routine use of viscoelastic hemostatic assays (rotational thromboelastography (TEG) or rotational thromboelastometry). A single randomized, controlled trial of TEG-guided transfusion in cirrhotic patients with coagulopathy (INR > 1.8 and/or platelet count <50 × 10 9 /L) undergoing invasive procedures found a significant reduction in blood product administration with TEG compared to the standard lab value–based group. However, only half of the patients enrolled in the trial were undergoing procedures associated with greater than 3% risk of hemorrhage.
With respect to pain management, thoracic epidural analgesia is a highly effective means of pain control for hepatic resection. Although its use for this purpose has been described in a number of case series, it remains controversial due to the development of postoperative coagulopathy and thrombocytopenia following hepatic resection and the associated risk of epidural hematoma. Postoperative coagulopathy and thrombocytopenia occur in 21% to 100% of patients undergoing liver resection, depending on the volume of tissue resected. The INR peaks around postoperative day (POD) one or two while the platelet count is lowest on POD three or four. The values typically return to baseline by POD four or five; however in some patients they may remain elevated for up to a week, leading to delays in epidural catheter removal or the decision to transfuse FFP to facilitate catheter removal. An additional concern is the accidental dislodgment of the epidural catheter while the patient is coagulopathic which has been reported to occur in up to 7% of patients. The degree of coagulopathy is influenced by the extent of the resection. In a review of 759 patients undergoing hepatic resection, the incidence of coagulopathy was 39.1% in patients who had undergone major hepatectomies (>2 segments) versus 21.3% in patients who had undergone minor hepatectomies (≤2 segments). Other factors that are independently associated with postoperative coagulopathy included preexisting cirrhosis, preoperative INR ≥ 1.3, preoperative platelet count <150,000/μL, estimated blood loss ≥ 1000 mL, and the duration of surgery. Although no case series has reported the complication of an epidural hematoma, epidural hematoma is quite rare and the numbers of published cases may have been inadequate to detect its occurrence.
Rapid sequence induction should be considered in patients with significant ascites. Maintenance of general anesthesia has been described with both volatile anesthetics and intravenous anesthetics. There is no compelling clinical evidence for the benefit of one specific agent over another. Regardless of the approach, anesthetic dosing should be titrated to the patient’s requirements, taking into account the pharmacokinetics and pharmacodynamics of common anesthetic agents in patients with liver disease. The anesthesiologist should understand the vascular occlusion strategies that may be employed during the operation along with their hemodynamic effects. The Pringle maneuver, both during open and laparoscopic hepatic resections, leads to an increase in MAP and systemic vascular resistance and a decrease in CO. During open hepatic resection, portal triad clamping leads to a decrease in left ventricular (LV) end-diastolic area (i.e., LV preload). During laparoscopic hepatic resection, in the presence of pneumoperitoneum, PTC also increases LV end-systolic wall stress (i.e., LV afterload).
Enhanced Recovery After Surgery and Liver Resection
A number of randomized controlled trials have been conducted comparing the use of enhanced recovery after surgery (ERAS) protocols with traditional management in patients undergoing both open and laparoscopic liver resections. Although ERAS Society consensus guidelines exist, these trials differed with respect to the number and nature of ERAS elements. Most incorporated preoperative education, early enteral nutrition, and early mobilization. The implementation of an ERAS protocol for liver resection surgery has been shown to reduce minor adverse events, hospital length of stay, hospital cost, and to improve patient quality of life. A metaanalysis published by the Cochrane group found that the use of ERAS protocols resulted in a reduction in mild adverse events, hospital length of stay, and cost. However, all studies were at high risk of bias and the quality of available evidence was determined to be low.
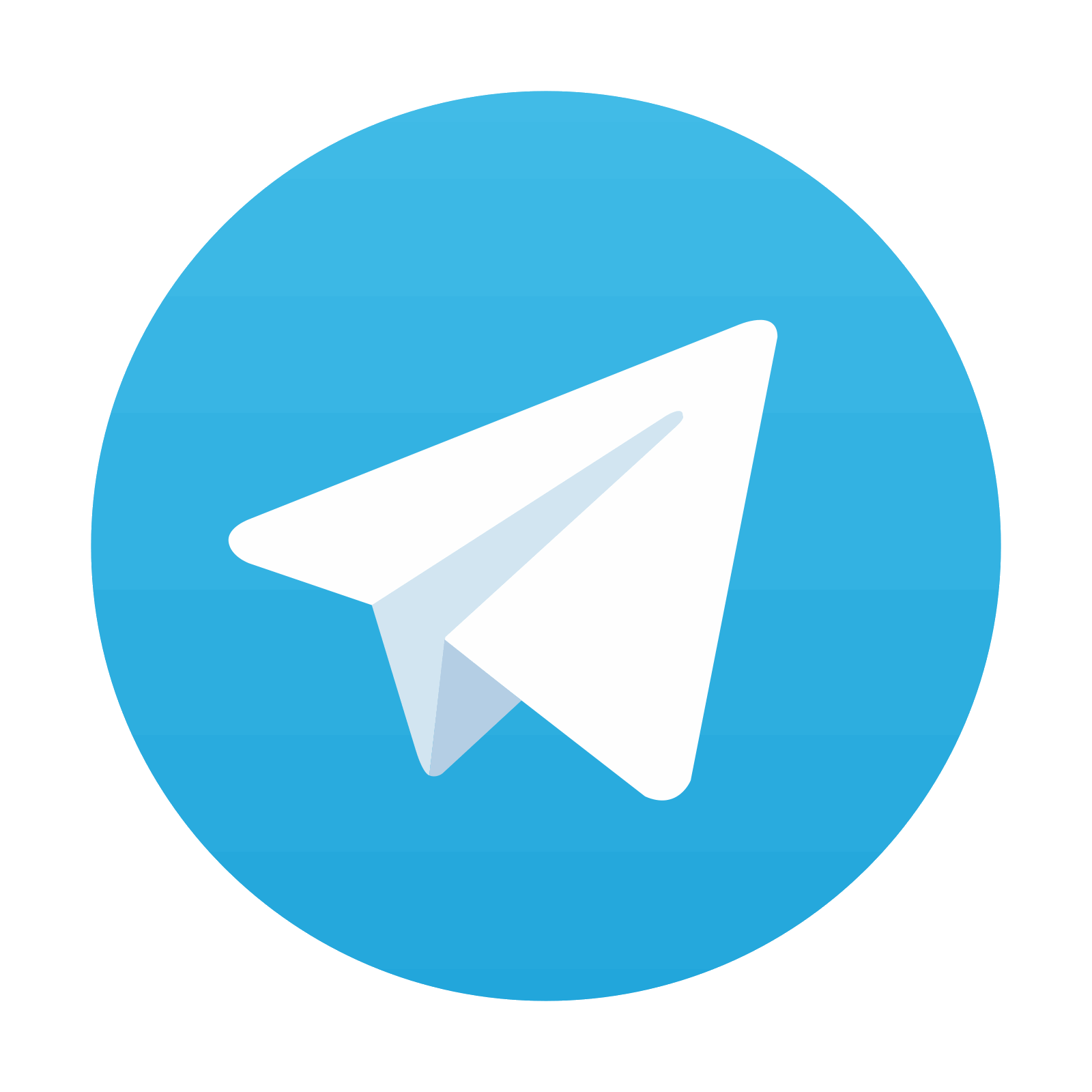
Stay updated, free articles. Join our Telegram channel
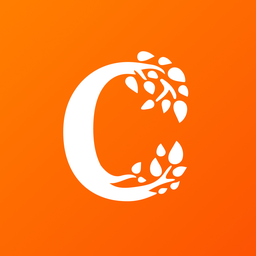
Full access? Get Clinical Tree
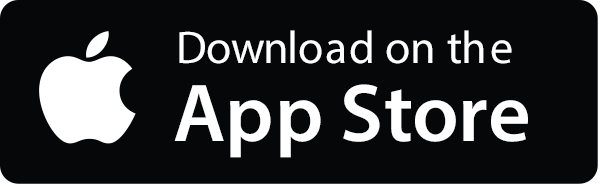
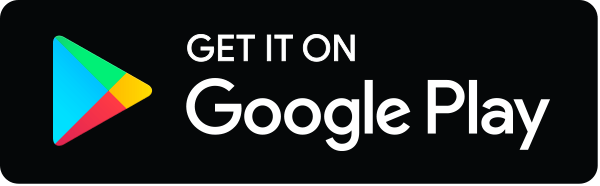