The [Na+] is multiplied by 2 to account for the accompanying anions (mostly chloride and bicarbonate) that provide electroneutrality. The corrections in the glucose concentration and BUN are to convert mg/dL into mmol/L.
The plasma osmolality is primarily determined by the concentration of sodium salts with minor contributions from glucose and BUN. As BUN is lipid soluble and equilibrates across the cell membranes, it is an ineffective osmole and does not contribute to fluid distribution, and therefore it is omitted from calculation from effective plasma osmolality as follows:
Effective serum osmolality:
Be advised, however, that the calculation introduces a bias, overestimating osmolality in the lower ranges and underestimating it in the higher ranges [4]. Hence, the single most important factor is the serum sodium concentration.
CLINICAL PEARL
Administration of large volumes of isosmolar crystalloids will dilute plasma protein concentration and result in peripheral edema, but will not generally increase brain water content (i.e., cerebral edema) or ICP.
C. Colloid oncotic pressure (COP). Osmolarity and osmolality are determined by the total number of dissolved “particles” in a solution, regardless of their size. COP is the osmotic pressure produced by large molecules (e.g., albumin, hetastarch, dextran). This factor becomes particularly important in biologic systems in which vascular membranes are often permeable to small ions but not to large molecules (typically plasma proteins). In such situations, proteins might be the only osmotically active particles. Normal COP is ≅20 mm Hg (or equal to ≅1 mOsm/kg).
1 2
D. Starling’s hypothesis. In 1898, Starling published his equations describing the forces driving water across capillary endothelium. The major factors that control the movement of fluids between the intravascular and extravascular spaces are the transcapillary hydrostatic gradient, the osmotic and oncotic gradients, and the relative permeability of the capillary membranes that separate these spaces. The Starling equation is as follows:
where FM is fluid movement, k is the filtration coefficient of the capillary wall (i.e., how leaky it is), Pc is the hydrostatic pressure in the capillaries, Pi is the hydrostatic pressure (usually negative) in the interstitial (extravascular) space, and pi and pc are interstitial and capillary osmotic pressures, respectively.
Fluid movement is, therefore, proportional to the hydrostatic pressure gradient minus the osmotic pressure gradient across a capillary wall. The magnitude of the osmotic gradient depends on the relative permeability of the vessels to solute. In the majority of the capillary beds in the human body (the periphery), the configuration of the desmosomes that connect endothelial cells creates an effective intercellular pore size of 65 Å. Small electrolyte molecules (Na+, Cl−) pass freely, while large molecules such as proteins (Fig. 3.1) cannot cross the membrane. As a result, p is defined only by colloids, and the Starling equation can be simplified by saying that fluid moves into a tissue whenever either the hydrostatic gradient increases (either intravascular pressure rises or interstitial pressure falls) or the osmotic gradient decreases.
FIGURE 3.1 Schematic diagram of a peripheral capillary. The vessel wall is permeable to both water (H2O) and small ions, but not to proteins (P).
FIGURE 3.2 Schematic diagram of a cerebral capillary. The blood-brain barrier (BBB) is impermeable to small ions and proteins (P), but not to water (H2O).
In normal situations, the intravascular protein concentration is higher than the interstitial concentration, acting to draw water back into the vascular space. If COP is reduced (e.g., by dilution with large amounts of isotonic crystalloid), fluid begins to accumulate in the interstitium, producing edema (e.g., marked peripheral edema in patients given many liters of crystalloid during surgery or resuscitation). By contrast, the blood–brain barrier (BBB) is impermeable to both ions and proteins so that osmotic pressure is determined by the total osmotic gradient, of which COP contributes only a tiny fraction (≅1 mOsm/kg) (Fig. 3.2).
E. Interstitial clearance. Peripheral tissues have a net outward movement of fluid (i.e., the value of FM is positive). Edema is not normally present, however, because this extravasated fluid is cleared by the lymphatics. While many researchers agree that there is some lymphatic drainage of the brain, most interstitial fluid in the brain is cleared either by bulk fluid flow into the cerebrospinal fluid (CSF) spaces or via pinocytosis back into the intravascular compartment. This is a slow process and probably does not counteract rapid fluid movement into the interstitial space.
F. Hydrostatic forces and interstitial compliance. In the tissues, the net hydrostatic gradient is determined by (a) intravascular pressure and (b) interstitial tissue compliance. Normally, the direction is outward (capillary to interstitium). There is no question that in the brain (or in any organ), elevated intravascular pressure, such as that produced by either high jugular venous pressure or a head-down posture, can increase edema formation. However, an often overlooked factor that influences the pressure gradient is the interstitial compliance (i.e., the tendency of tissue to resist fluid influx). The loose interstitial space in most peripheral tissues does little to impede the influx of fluid. This explains the ease with which edema develops around, for example, the face and the eyes, even with minor hydrostatic stresses (e.g., a face-down posture). By contrast, the interstitial space of the brain is extremely noncompliant, resisting fluid movement. As a result, minor changes in driving forces (either hydrostatic or osmotic/oncotic) do not produce measurable edema. However, a vicious cycle can develop so that, as edema forms in the brain, the interstitial matrix is disrupted, the compliance increases, and additional edema forms more easily. In contrast, the closed cranium and ICP can act to retard fluid influx. This may partially explain the exacerbation of edema formation that can occur after rapid decompression of the intracranial space.
1 2
G. BBB and serum osmolality. The endothelial cells of the cerebral microvasculature differ from other endothelial phenotypes by the absence of fenestrations and sparse pinocytic vesicular transport. They are held together by continuous tight junctions, form the BBB, and exhibit specific protective properties. The effective pore size of the BBB is only 5 to 7 Å, making this unique structure normally impermeable to all large hydrophilic molecules (e.g., plasma proteins and synthetic colloids, such as hetastarch and dextrans) and relatively impermeable to many small polar solutes (Na+, K+, Cl−). The BBB functions as a semipermeable membrane that allows only water to move freely between the interstitial space and the vasculature (Fig. 3.2), according to osmotic gradients.
The reduction of serum osmolality (e.g., by infusing water or large volumes of nonisotonic crystalloid solution) increases brain water content [5]; conversely, the increase of osmolality reduces brain water content [6]. Even small changes can produce measurable changes in brain water content: In experimental animals, a reduction in plasma osmolality of as little as 5%, under otherwise normal conditions, causes brain edema and increases ICP [5].
2
H. BBB and COP. COP contributes to only a tiny fraction of the total osmolality (≅1 mOsm/kg) and, when the BBB is intact, can be responsible for only a small driving force. Normal plasma COP is approximately 20 mm Hg, whereas that in the brain interstitium is approximately 0.6 mm Hg. This is equal to the force that could be generated by a change in the capillary/tissue osmotic gradient of only 1 mOsm/kg. We would therefore predict that changes in COP have only minimal effect on brain water content. Several animal experiments have demonstrated that normal brain water content can be altered by small changes in osmolality but not by clinically achievable changes in COP [7]. There was no increase in brain water content (i.e., edema) in regions with an intact BBB, even after a reduction of approximately 50% in COP. If the BBB becomes permeable to both small and large molecules (i.e., complete breakdown of the BBB as is common with several clinical injuries), it is almost impossible to maintain any form of osmotic or oncotic gradient between the intravascular compartment and the brain interstitium. As a result, no changes in brain water content would be expected with a change in either gradient. Indeed, in several animal models resembling human brain injuries (e.g., implanted glioma and freezing lesion, experimental model of brain tumor and trauma, respectively), edema induced by a reduction in total serum osmolality occurred only in normal regions of the brain relatively distant from the focus of injury. In keeping with this, several studies have shown that acute hyperosmolality (as with mannitol, urea, glycerol, hypertonic saline [HS]) reduces water content only in normal brain tissue where the BBB is intact. Conversely, when the BBB is severely damaged, investigations have failed to demonstrate that reducing the COP affects brain edema [8]. In the presence of mild injury to the BBB in experimental animals, a reduction in COP may potentially aggravate brain edema. Therefore, it is possible that, with a less severe injury, the BBB may function similarly to the peripheral tissue [7]. In summary, injury to the brain interferes with the integrity of the BBB to varying degrees, depending on the severity of the damage. Regions where there is a complete breakdown of the BBB, there will be no osmotic/oncotic gradient. Water accumulation (i.e., brain edema) occurs because of the pathologic process itself and cannot be directly influenced by the osmotic/oncotic gradient. In other regions where there is moderate injury to the BBB (i.e., a mild opening rendering pore size similar to the periphery), it is possible that the colloid oncotic gradient is effective as in the peripheral tissue. Finally, the BBB is normal in a significant portion of the brain. The presence of a functionally intact BBB is essential if osmotherapy is to be effective.
II. Fluids for intravenous administration. The physicians can choose from among a variety of solutions suitable for intravenous use, categorized mostly on the basis of osmolality, oncotic pressure, and dextrose content (Tables 3.1 and 3.2).
Table 3.1 Composition of commonly used intravenous fluids: crystalloids
Table 3.2 Composition of commonly used intravenous fluids: colloids
A. Crystalloids are solutions of inorganic ions and small organic molecules dissolved in water, do not contain high–molecular-weight compounds and have an oncotic pressure of zero (Table 3.1). Crystalloids are inexpensive, require no special compatibility testing, and have a very low incidence of adverse reactions and no religious objections to their use. Crystalloids can be hypo-osmolar, isosmolar, or hyperosmolar with respect to plasma. Crystalloids with an ionic composition close to that of plasma may be referred to as “balanced” or “physiologic.”
1. Hypo-osmolar crystalloids, especially in large amounts, reduce plasma osmolality, drive water across the BBB, cause brain edema even in entirely normal brain, and increase ICP. Whatever fluid regimen is chosen it should not have sufficient free water to cause reduction in osmolality. Five percent dextrose (D5W) is essentially water (as the sugar is metabolized very quickly), provides “free water” which disperses throughout the intracellular and extracellular compartments, and has little use as a resuscitative fluid. Hypo-osmolar crystalloids (0.45% NaCl or D5W) should be avoided in neurosurgical patients.
2. Isosmolar crystalloids such as normal saline (NS, 0.9% NaCl) and Plasmalyte have an osmolarity =300 mOsm/L, and do not change plasma osmolality. Potassium, calcium, and lactate may be added to more closely replicate the ionic makeup of the plasma (Table 3.1).
Lactated Ringer’s solution is slightly hypotonic (measured osmolarity =254 mOsmol/kg) in relation to the plasma [1,3], and can decrease serum osmolality and increase brain water content and ICP, mostly when large amounts are infused. Small volumes of lactated Ringer’s (1 to 2 L) are unlikely to be detrimental and can be used safely, for example, to compensate for the changes in venous capacitance that typically accompany the induction of anesthesia. If large volumes are needed (either to replace blood loss or to compensate for some other source of volume loss), a change to a more isotonic fluid such as normal saline is probably advisable. It is also important to remember that rapid infusion of large volumes of isotonic saline can induce a dose-dependent dilutional–hyperchloremic acidosis. There is debate about the morbidity associated with this condition, and it is suggested that dilutional–hyperchloremic acidosis is a benign phenomenon, which usually requires no treatment, but must be differentiated from other causes of metabolic acidosis [9].
Lactated Ringer’s solution and Plasmalyte contain bicarbonate precursors. These anions (e.g., lactate) are the conjugate base to the corresponding acid (e.g., lactic acid) and do not contribute to the development of acidosis, as they are administered with Na+ rather than H+ as the cation. The metabolism of lactate in the liver results in the production of an equivalent amount of bicarbonate.
3
3. Hyperosmolar crystalloids. Mannitol and HS. Solutions can be made hyperosmolar by the inclusion of electrolytes (e.g., Na+ and Cl−, as in HS) and low–molecular-weight solutes such as in mannitol (MW 182) (Table 3.1). Osmotic therapy is a cornerstone in management of ICP induced by cerebral edema. Its effectiveness depends on the integrity of the BBB, the reflection coefficient of the osmotic agent, and the osmotic gradient created.
a. Mannitol. Mannitol is the primary osmotic drug for the control of increased ICP [10], and is recommended as drug of choice by both the Brain Trauma Foundation and the European Brain Injury Consortium. The recommended dose of mannitol is 0.25 to 1 g/kg, and the smallest possible dose is selected and infused over 10 to 15 minutes. The mechanism through which mannitol acts is still unknown. Very recent human PET studies do not support the hypothesis of autoregulatory cerebral vasoconstriction induced by volume expansion or by changes in blood viscosity [11]. They suggest that the ability of mannitol to lower ICP and reduce mass effect may be better explained by a reduction in brain water content, since mannitol establishes an osmotic gradient between blood and brain in the presence of a relatively intact BBB. Mannitol has several limitations. It might increase circulatory blood volume from hyperosmolality, and although this phenomenon does not occur when mannitol is given at moderate doses, care should be taken when it is given to patients with congestive heart failure. Hyperosmolality is a common problem, and a serum osmolality >320 mOsm/L is associated with adverse renal and CNS effects. The osmotic diuresis may lead to hypotension, especially in hypovolemic patients, and, although controversial, the accumulation of mannitol in cerebral tissue can reverse the brain–blood gradient with exacerbation of the edema and increased ICP. Often furosemide is used in conjunction with mannitol. In animals furosemide enhances the effect of mannitol on plasma osmolality, resulting in a greater reduction of brain water content [12]. This effect, with the decreased CSF production induced by furosemide, can explain the synergistic effect of mannitol and furosemide on intracranial compliance. In the clinical practice, the benefit of osmotic treatment with furosemide is likely to be small, if it exists at all.
b. Hypertonic Saline. In humans, acute resuscitation from hemorrhagic shock with sodium-based hypertonic solution (HS) is associated with improved outcome in patients with multiple trauma and head injuries [13]. Several randomized clinical trials have suggested that HS may be superior to mannitol in reducing ICP [14]. HS is potentially more effective because the permeability of the BBB to Na+ is low, and the reflection coefficient (selectivity of the BBB to a particular substance) of NaCl is more than that of mannitol. Moreover, HS solutions result in significant volume expansion, improved cardiac output, improved regional blood flow, and beneficial immunomodulation. A recent clinical trial has been unable to demonstrate improvement in 6-month neurologic outcome or survival in head trauma patients without evidence of shock, suggesting that HS may be better in hypotensive, brain-injured patients [15]. There is no question that HS can quickly restore intravascular volume while reducing ICP through brain water reduction in uninjured brain [6]. In animal models of head trauma, HS improves systemic hemodynamics and cerebral blood flow (CBF) and may enhance cerebral microcirculation by reducing the adhesion of polymorphonuclear cells and stimulating local release of nitric oxide. In both pediatric and adult traumatic brain injury (TBI) patients, HS has been used effectively to reduce elevated ICP unresponsive to mannitol treatment [16].
Adverse effects after HS administration include renal failure, coagulopathy, hyperkalemia, pulmonary edema, and central pontine myelinolysis. The evidence for renal failure, coagulopathy, pulmonary edema, and hyperkalemia is tenuous, and it must be noted that central pontine myelinolysis has never been reported in human studies. Magnetic resonance imaging and postmortem studies failed to demonstrate central pontine myelinolysis despite maximum sodium levels of 182 mEq/L [16]. In neurosurgical patients undergoing elective supratentorial procedures, we have shown that equal volumes of 20% mannitol and 7.5% HS reduce brain bulk, as assessed by the neurosurgeon, and CSF pressure to the same extent (Fig. 3.3); serum sodium, however, increased during the HS administration and peaked at more than 150 mEq/L at the conclusion of the infusion (Fig. 3.4) [17]. The sodium load may be a concern in patients with neurologic injury and/or at risk for seizures.
FIGURE 3.3 Cerebrospinal fluid pressure (CSFP) in patients treated with equal volume of 7.5% hypertonic saline (HS) and 20% mannitol (M), subdivided according to baseline CSFP (< or >15 mm Hg).
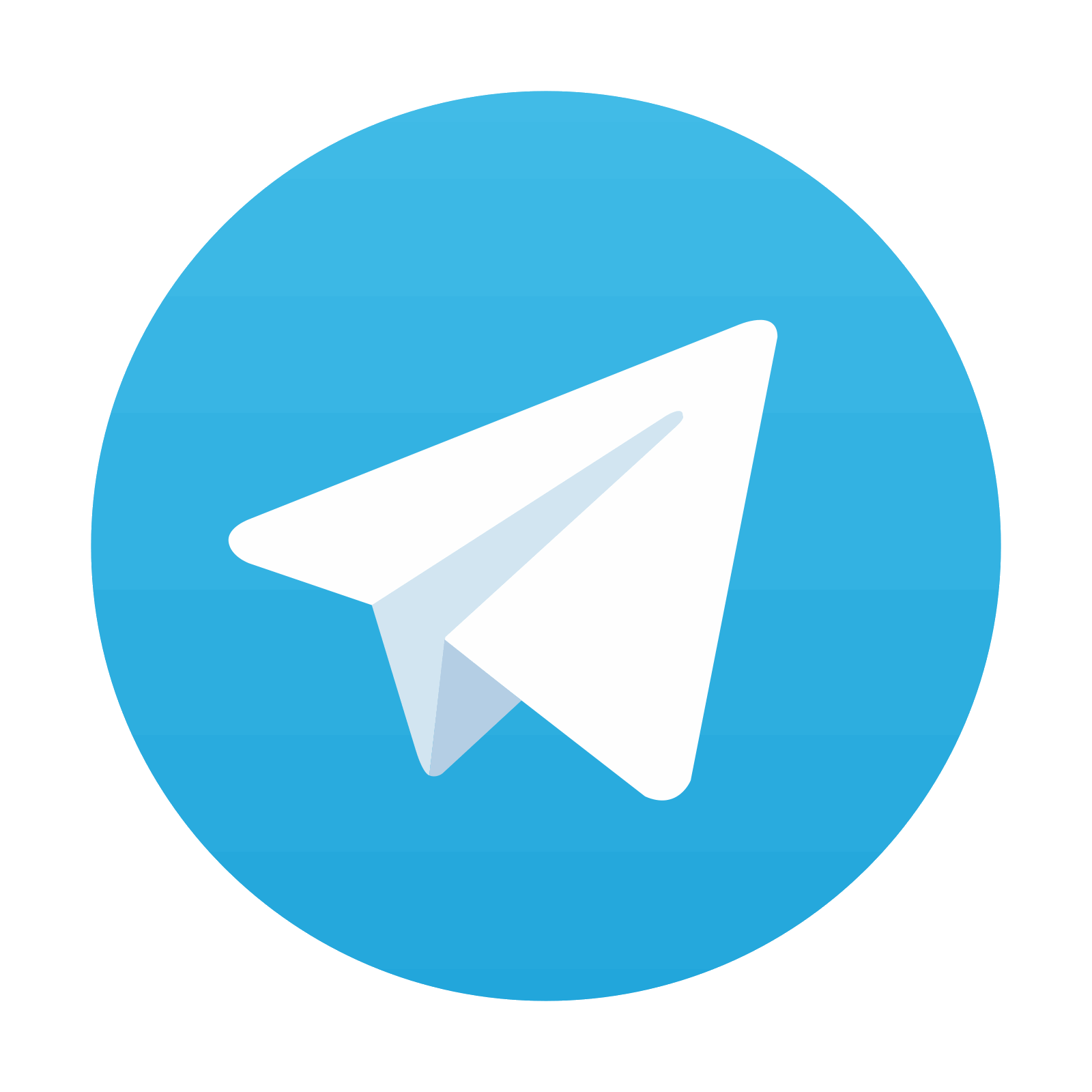
Stay updated, free articles. Join our Telegram channel
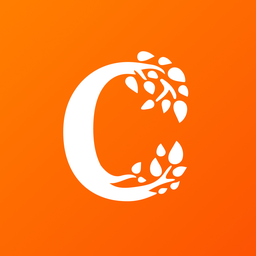
Full access? Get Clinical Tree
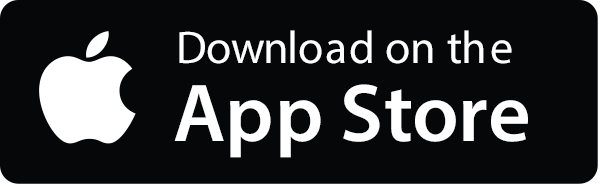
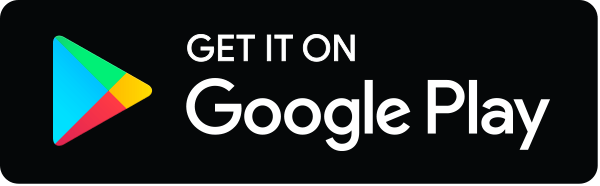