Critically ill patients have disturbances in multiple organ systems and hence are at risk for serious imbalances in electrolyte and water handling. Electrolyte disorders are extremely prevalent in the ICU: Hyponatremia can be found in 18% of ICU patients,1 hypokalemia in 21%,2 hypomagnesemia in more than 50%,3 hypocalcemia in 20%,4 and hypophosphatemia in 28%.5 This chapter will outline these disturbances with an emphasis on pathophysiology and treatment of electrolyte problems more commonly seen in the intensive care unit.
Plasma sodium (Na) concentration is the principal determinant of the relative volume of extracellular fluid.6 It is the major extracellular solute, whereas potassium is the major intracellular solute. Total solute activity, also called osmotic activity and expressed in osmoles (osm), is the sum of the individual osmotic activities of all the solute particles in the solution. For monovalent ions, the osmotic activity in milliosmoles (mOsm) per unit volume is equivalent to the concentration of the ions in milliequivalents (mEq) per unit volume.
Example: Osmotic activity in isotonic saline (0.9% NaCl)
Osmolarity is the osmotic activity per volume of solution, whereas osmolality is the osmotic activity per kilogram of water (mOsm/kg H2O). Osmolality is used to describe the osmotic activity of body fluids. Since the weight of body fluids is basically equal to the weight of water, there is little difference between osmolality and osmolarity of body fluids. The effective osmolality or osmotic activity in 2 solutions is called tonicity. Water passes from the solution of lower osmotic activity to the solution of higher osmotic activity. The tendency for water to move into and out of cells is determined by the relative tonicity of intracellular and extracellular fluids. Because of the ability of water to pass freely across membranes, the intracellular and extracellular space have equivalent osmolality, normally about 280 mOsm/kg (Fig. 2-1). However, the intracellular space comprises a larger percentage of total body water (about 60%).
Urea is freely permeable across cell membranes. An increase in the urea concentration in extracellular fluid increases the osmolality of the extracellular fluid but does not increase the tonicity of the extracellular fluid or cause a net movement of water out of cells. Azotemia (increase in blood urea nitrogen [BUN]) is a hyperosmotic condition but not a hypertonic condition.
Major solutes in extracellular fluid include sodium, chloride, glucose, and urea. Plasma osmolality is estimated by the following equation:
Solutes other than sodium, chloride, glucose, and urea are present in extracellular fluid, so measured plasma osmolality will be greater than calculated plasma osmolality; this osmolar gap is normally as much as 10 mOsm/kg H2O. An increase in the osmolar gap occurs when certain toxins (ethanol, methanol, ethylene glycol, or unidentified toxins that accumulate in renal failure) are in the extracellular fluid.
Most hyponatremic states, defined as Na less than 135 mEq/L, are secondary to hypotonic, low osmolality conditions and are classified based on extracellular fluid volume status. Intravascular volume is not easily measured, which necessitates clinical determination by history, physical exam, and laboratory results.7 Three laboratory tests necessary in the workup of hyponatremia are serum osmolality, urine osmolality, and urine sodium.
Isotonic hyponatremia is defined as a serum osmolarity of 280 to 295 mOsm. The differential includes distinguishing pseudohyponatremia from hypertriglyceridemia, hyperglobulinemia, and hyperproteinemia (> 10 g/dL). Hypertonic hyponatremia is defined as serum osmolarity of greater than 295 mOsm. The differential includes hyperglycemia, mannitol, sorbitol, glycine, and intravenous immunoglobulin (IVIG). Hypotonic hyponatremia is defined as serum osmolarity of less than 280 mOsm.
Further characterization of hypotonic hyponatremia is dependent on evaluation of urine osmolality and urine sodium. Hypovolemic hypotonic hyponatremia is suggested by urine osmolality greater than 450 mOsm/kg. Urine sodium of less than 20 mEq/L suggests vomiting, diarrhea, third space loss, trauma, or sweating. Urine sodium greater than 20 mEq/L suggest diuretics, mineralocorticoid deficiency, salt wasting nephropathy, renal tubular acidosis, metabolic alkalosis, cerebral salt wasting syndrome, and osmotic diuresis. Hypervolemic hypotonic hyponatremia is suggested by urine osmolality of greater than 100 mOsm/kg. Urine sodium of less than 20 mEq/L or greater than 20 mEq/L if recently diuresed would suggest congestive heart failure (CHF), cirrhosis, nephrotic syndrome, low arterial volume, and low aldosterone. A urine osmolality greater than 100 mOsm/kg indicates impaired ability of the kidneys to dilute urine, usually secondary to elevated levels of antidiuretic hormone (ADH). Urine sodium is usually low due to secondary renal sodium conservation resulting from activation of the renin-angiotensin-aldosterone system despite total body volume overload. A urine sodium level greater than 20 mEq/L suggests renal failure.
Euvolemic hypotonic hyponatremia has a serum osmolarity of less than 280 mOsm and no edema. A urine osmolarity of greater than 100 mOsm/kg with a urine sodium level greater than 20 mEq/L suggests hypothyroidism, glucocorticoid deficiency, syndrome of inappropriate antidiuretic hormone secretion (SIADH), stress, or drug use. A urine osmolarity of less than 100 mOsm/kg with a urine sodium level less than 20 mEq/L suggests primary polydipsia and low solute intake like beer potomania.
Treatment of hyponatremia is dependent on duration (acute [≤ 48 hours] versus chronic [> 48 hours]), symptoms, and severity. Hyponatremia is presumed chronic (unless there is clear evidence to the contrary), hence, cerebral symptoms ultimately determine the treatment of hyponatremia. Symptoms include headache, nausea, vomiting, fatigue, gait disturbance, confusion, seizures, obtundation, coma, and respiratory arrest. Mild hyponatremia (Na 130–134 mEq/L) generally requires a less aggressive therapeutic approach than severe hyponatremia (Na ≤ 120 mEq/L).8-12 In most cases, the rate of rise of serum sodium should not exceed 0.5 mEq/L/h and should not exceed 8 mEq/L in any given 24-hour period, or 18 mEq/L in 48 hours.
Emergent treatment is warranted with neurologic symptoms, and the goal is to increase serum sodium rapidly by 4 to 6 mEq/L over a period of 3 to 4 hours. To raise the serum sodium by 1.5 mEq/L in men and 2 mEq/L in women, give a 100-cc bolus of 3% saline over 10 minutes. If neurologic symptoms persist or worsen, boluses can be repeated twice.10-12 Plasma sodium should be checked in 2 to 3 hours initially and then every 3 to 4 hours until the patient is stable.
Correction begins with calculation of sodium deficit, as determined by the formula:
Normal total body water (TBW) is approximately 60% lean body weight of men and 50% lean body weight of women. A 3% sodium chloride solution contains 513 mEq of sodium per liter and a 0.9% sodium chloride solution contains 154 mEq of sodium per liter.
The rationale for the goals of treatment begins with osmolytes. Osmolytes are small molecules, such as glutamate and taurine, that can be synthesized or transported out of the cell to help prevent osmotic damage to neurons. Under osmotic stress (increases or decreases in serum sodium), neurons are capable of altering their solute concentrations by changing osmolyte concentration. Normally, during hyponatremia, osmolytes will be transported out of the cell to prevent water from diffusing into the neuron, thereby preventing an increase in cell volume. These protective mechanisms take time to occur. Astrocytes will take 24 to 48 hours to release sufficient osmolytes to restore cell volume to normal. However, loss of these osmolytes will leave the astrocyte susceptible to hypertonic injury. The rapid correction of hyponatremia will lead to apoptosis of astrocytes, causing osmotic demyelination.8 This syndrome is associated with progressive quadriplegia, dysphagia, dysarthria, and alterations in consciousness that occur days after treatment. This underscores the need to correct chronic (occurring over > 48 hours) hyponatremia more slowly, whereas acute hyponatremia may be corrected more quickly since astrocytes have not had sufficient time to release osmolytes. Disease states that increase the risk of osmotic demyelination include alcoholism, liver failure, malignant disease, hypokalemia, serum sodium less than 120 mEq/L, and malnutrition.
There are no controlled trials validating overcorrection treatment strategies, although experimental studies in animals support that lowering serum sodium prevents osmotic demyelination.13 In addition, a small series of patients with hyponatremia who have overcorrected has demonstrated that lowering serum sodium is well tolerated.14 Expert panels recommend lowering serum sodium to goal levels for patients who are at high risk for osmotic demyelination and who have overcorrected at the 24-hour mark. They recommend that sodium should be lowered by replacing free water urinary losses with 5% dextrose in water (D5W) or by giving 2 to 4 μg of desmopressin intravenously (IV) every 8 hours with boluses of 3 mL/kg D5W over 1 hour and rechecking serum sodium after each bolus.7 For patients with chronic hyponatremia in clinically euvolemic and hypervolemic states, vasopressin receptor antagonists can be utilized but must be initiated in the hospital setting. Conivaptan is a combined vasopressin 1a receptor (V1aR) and V2R receptor antagonist available only as IV formulation and initiated with a 20-mg bolus over 20 minutes and then 20 to 40 mg/day. Adverse effects include headache, thirst, and hypokalemia. It should not be used for more than 4 days because of drug-interaction effects with other medications metabolized by the hepatic CYP3A4 enzymes.7 Tolvaptan, an oral V2R antagonist, is initiated at 15 mg and then titrated to a maximum of 60 mg/day if the sodium level remains less than 135 mEq/L. Side effects include dry mouth, increased urinary frequency, thirst, dizziness, and orthostatic hypotension.
Vaptans are not indicated for hypovolemic hyponatremia because simple volume expansion would be expected to increase sodium levels secondary to decreased vasopressin release and resultant aquaresis. In addition, these agents will likely not be effective in patients with a creatinine level greater than 2.5 mg/dL. They should be avoided in patients with underlying liver disease or cirrhosis due to the potential of inducing further liver injury. In 1 trial of polycystic kidney disease patients treated with tolvaptan, a significant number of patients developed elevations of the liver enzyme alanine aminotransferase (ALT) of 3 times normal levels.7
Hypernatremia, defined as a sodium concentration greater than 145 mEq/L, commonly occurs secondary to free water loss in excess of sodium loss. This can be seen in diabetes insipidus, from hypotonic fluid losses such as those that occur in gastrointestinal loss secondary to osmotic diarrhea, or in osmotic diuresis.15 Rarely, it can occur because of excessive sodium intake in relation to free water intake (usually iatrogenic). The state of intravascular volume can be used as a reflection of the state of extracellular volume.
Patients with acute hypernatremia can exhibit symptoms that include lethargy, weakness, irritability, seizures, and coma. In those with chronic hypernatremia, lasting more than 48 hours, fewer symptoms occur because of osmotic adaptation.
Signs of hypernatremia are usually exhibited as volume depletion and include dry mucous membranes, decreased skin turgor, postural hypotension, and jugular venous pressure less than 5 cm H2O. In patients with heart failure taking loop diuretics, signs of volume expansion can be seen, including peripheral edema and pulmonary edema.
It is important to remember that all patients with hypovolemia, regardless of serum sodium concentration, should be administered isotonic crystalloid solutions first to improve tissue perfusion. After hypovolemia is corrected with isotonic fluids (normal saline or lactated Ringer solution), the next step is to calculate and replace the free water deficit using the following formula:
Normal TBW is usually 60% of lean body weight in men and 50% of lean body weight in women. However, in hypernatremia associated with free water deficits, normal TBW should be approximately 10% less than usual:
In patients with hypovolemic hypernatremia, free water deficits should be replaced slowly, over 48 to 72 hours, to prevent cerebral edema. Generally, one-half of TBW deficit is replaced in the first 24 hours and the rest over the next 48 hours. Replacement should include insensible water losses, and frequent monitoring of sodium and other electrolytes should be performed over the first 24 hours.
Ninety-eight percent of potassium is located intracellularly, with 2% found extracellularly. This large gradient is a major determinant for setting the thresholds of cellular action potentials, mainly in the cardiac and neuromuscular cells.16 Maintaining a distribution of potassium in the extracellular fluid within the narrow margin of 3.5 to 5.5 mEq/L is critical for normal cell function.17 This homeostasis is maintained by the sodium-potassium—adenosine triphosphatase (Na-K-ATPase) pump in the cell membrane,18 and the total body potassium content is primarily preserved by the kidneys (Fig. 2-2). Disruption of renal function or the action of the Na-K-ATPase pump can lead to hyperkalemia or hypokalemia.
FIGURE 2-2
The sodium-potassium–adenosine triphosphatase (Na-K-ATPase) pump. Three sodium ions are moved out of the cell while 2 potassium ions are moved intracellularly. Adenosine triphosphate hydrolysis is required for each action of the pump. The result is a slightly negative intracellular charge and slightly positive extracellular charge.

The approach to evaluating a patient with hyperkalemia requires evaluation of each of the following possibilities:
laboratory error and pseudohyperkalemia
potassium shift from intracellular to extracellular
decreased renal excretion of potassium
An electrocardiogram (ECG) is recommended as soon as a hyperkalemia is suspected.16 As hyperkalemia worsens, impulse generation is suppressed, resulting in bradycardia, conduction blocks, and ultimately cardiac arrest.19
Pseudohyperkalemia is an elevation in the measured serum potassium concentration due to potassium movement out of the cells (in vitro) without the true effect on cells.20 This should be suspected when there is no obvious cause for hyperkalemia in an asymptomatic patient who has no electrocardiographic manifestations of hyperkalemia. Examples of pseudohyperkalemia include prolonged specimen storage, hemolysis during venipuncture, repeated fist clenching and a tight tourniquet, thrombocytosis, and leukemoid reaction with white blood cell (WBC) count greater than 50 × 103/mL.18
Potassium shift from the intracellular to extracellular compartment can be seen in insulin deficiency, metabolic acidosis, b-adrenergic antagonists, drugs that inhibit Na-K-ATPase, and hyperkalemic periodic paralysis. Acute cell tissue breakdown (eg, tumor lysis syndrome, rhabdomyolysis, or massive transfusion) causes potassium to be released into the extracellular space.21,22
Decreased renal excretion of potassium can be due simply to fall in glomerular filtration rate (GFR), which leads to decreased glomerular filtration of potassium, and hence reduced excretion. Even if GFR remains normal, potassium excretion may be reduced considerably if potassium secretion is impaired at the cortical collecting duct. At this section of the tubule, sodium diffuses through the electroneutral sodium channel (ENaC) and down its concentration gradient. Potassium will then diffuse out of the cell, down its concentration gradient, and into the tubule lumen, in order to maintain electroneutrality (Fig. 2-3). Processes that interfere with this sodium-potassium transport cause hyperkalemia as well; examples of this are an impaired renin-aldosterone axis (eg, caused by angiotensin-converting enzyme [ACE] inhibitors and spironolactone), inhibition of ENaC function (caused by amiloride), and decreased distal tubular sodium delivery and urine flow (in acute kidney injury [AKI] and CHF).22 Table 2-1 outlines common medications that cause hyperkalemia.
FIGURE 2-3
Aldosterone diffuses across the cell membrane and binds the cytosolic mineralocorticoid receptor (M-R). Aldosterone binding to M-R leads to an increase in the number of open electroneutral sodium channels (ENaC) and increased activity of the sodium-potassium–adenosine triphosphatase (Na-K-ATPase) pump. Potassium diffuses into the tubule via a renal outer membrane potassium (ROMK) channel.

Mechanism | Medication |
---|---|
Potassium release from cells |
|
Inhibition of aldosterone production |
|
Inhibition or antagonism of effects of aldosterone |
|
Clinical effects of mild to moderate hyperkalemia are usually nonspecific and may include generalized weakness, fatigue, paresthesia, nausea, vomiting, intestinal colic, and diarrhea. Severe hyperkalemia may lead to life-threatening conditions such as cardiac blocks/arrhythmias and muscle weakness/paralysis.21
The ECG changes that occur with hyperkalemia are relatively characteristic and can sometimes point to hyperkalemia as etiology of symptoms before laboratory results are available. However, studies have shown that the ECG may not be sensitive to the severity of hyperkalemia in chronic cases.23 In general, the progression of ECG follows a pattern:24
Shortened QT and peaked T waves
Progressive lengthening of PR and QRS
Disappearance of P wave and development of a sine-wave pattern
In general, alterations in T-wave morphology and QT shortening occur when the serum potassium is above 6, whereas progressive lengthening of PR and QRS occur at higher serum potassium levels (> 7 mEq/L). However, one cannot predict ECG changes based on a given serum potassium level23,25 (and vice versa), as other factors affect cardiac conduction, such as associated hypocalcemia and hypomagnesemia. Patients with end-stage renal disease (ESRD) and chronic kidney disease (CKD) are less likely to manifest ECG changes with hyperkalemia, as it is thought that they manifest tolerance to hyperkalemia.26 In any event, the sign-wave pattern is an ominous sign and it will progress to asystole if not treated.
Hyperkalemia can be classified into the following 3 categories:16
mild: K+ = 5.5–6.5 mEq/L;
moderate: K+ more than 6.5–7.5 mEq/L; and
severe: K+ more than 7.5 mEq/L.
Stabilizing the cardiac membrane
Shifting potassium from extra- to intracellular space
Removing potassium from the body
Agent | Effect | Dose | Onset of Action | Duration of Treatment | Adverse Effect |
---|---|---|---|---|---|
Calcium gluconate (10% solution) | Stabilizes cell membrane | 1 g IV over 10 min | Immediate | 60 min, can be repeated | Arrhythmia |
Regular insulin | Intracellular shift | 10 units IV push (with 25 g of dextrose unless glucose ≥ 250) | 20 min | Lasts 4–6 h | Hypoglycemia within 1 h is very common (up to 75% of patients) |
Albuterol | Intracellular shift | 10 to 20 mg in 4 mL of saline by nebulization over 10 min | 30 min | 60 min | Tachycardia, arrhythmia |
Sodium bicarbonate | Intracellular shift | 150 mEq in 1 L of 5% dextrose in water over 2–4 h | Hours | Volume overload | |
Furosemide | Renal excretion | 40 mg every 12 h (may need to add saline if patient is not in heart failure) | 15 min | 6 h | Volume depletion, worsening azotemia |
Sodium polystyrene sulfonate | GI excretion | 15–30 g PO every 4–6 h | Unpredictable | Hours | Intestinal necrosis |
First, intravenous calcium is given if there are ECG changes, in order to stabilize the myocardial cell membrane by antagonizing excitation.16,21 Intravenous calcium is recommended in any patient with ECG changes or symptoms of hyperkalemia (muscle weakness or paralysis), or if potassium is 6.5 mEq/L or higher.26 Second, therapy should be given to shift potassium from extracellular to intracellular space in any patient with moderate to severe hyperkalemia (or ECG changes). Insulin with or without glucose and β2-adrenergic agonists (eg, salbutamol/albuterol) decrease serum potassium by stimulating the Na-K-ATPase pump, shifting potassium intracellularly in exchange for sodium.22 β2-Adrenergic stimulants combined with insulin have been shown to have an additive effect and also have been shown to reduce the incidence of insulin-induced hypoglycemia.27,18 Sodium bicarbonate, which causes a mild shift of potassium into cells due to a rise in systemic pH, is preferably given to patients who are acidotic. In hemodialysis patients with hyperkalemia, it has only a moderate effect if given as prolonged infusion.22 Overall, the efficacy of sodium bicarbonate for treatment of acute hyperkalemia is of modest benefit at most.
Third, total body potassium is reduced by other methods. In patients with normal to moderately impaired renal function, loop or thiazide diuretics can be used to increase renal excretion of potassium.18 Gastrointestinal cation exchange resins, such as sodium polystyrene sulfonate (SPS), bind potassium in the gastrointestinal tract in exchange for other cations, such as sodium or calcium. The efficacy of SPS in the treatment of hyperkalemia has been debated. A recent retrospective study suggests that SPS is effective in reducing potassium by 1 mEq/L but is associated with intestinal necrosis.22,28 Earlier studies have suggested that sorbitol or sorbitol mixed with SPS causes intestinal necrosis.23-27 Recent animal study have suggested SPS can cause intestinal necrosis alone.28 Despite this, SPS is still used in persistent hyperkalemia if a newer cation exchange resin such as patiromer is unavailable, other methods have failed to improve, and renal function is not restored. Patients in the postoperative setting and/or those who have gastrointestinal ileus or underlying bowel disease (eg, infection with Clostridium difficile) are at higher risk for intestinal necrosis and should not receive SPS. Patiromer is a newer cation exchange resin that has been studied for use in CKD patients with chronic hyperkalemia; it has not yet been studied for acute therapy of hyperkalemia, so it cannot be recommended for treatment of acute hyperkalemia.29 Renal replacement therapy (RRT) is the definitive treatment in severe hyperkalemia. Any patient with persistent hyperkalemia despite appropriate interventions described above should be initiated on hemodialysis. In addition, patients with severe AKI (creatinine 2–3 times baseline or oliguria) should be considered for urgent hemodialysis.26 Intermittent hemodialysis provides a substantially higher potassium clearance than continuous forms of RRT.22 Rebound hyperkalemia, due to extracellular shift of potassium from the intracellular compartment, can occur in 30% of patients an hour after intermittent hemodialysis, so repeat potassium should be checked a couple hours after completion of treatment.30
Hypokalemia is defined as a decreased level of potassium in the bloodstream (< 3.5 mEq/L). Hypokalemia generally occurs because of 1 of the following mechanisms:
poor oral intake,
intracellular potassium shift,
gastrointestinal losses, or
urinary losses.
Increased entry of potassium into the cell can lead to hypokalemia, although these effects are usually temporary and are most prominent in patients who are already deficient in total body potassium. As discussed above, insulin, albuterol, and dobutamine all increase the activity of the Na-K-ATPase pump and hence stimulate intracellular shift.32 A rise in the extracellular pH will also lead to a fall in serum potassium levels; in general, this fall is typically mild and the serum potassium concentration falls by less than 0.4 mEq/L for every 0.1 unit rise in pH.31
Severe hypokalemia due to intracellular shift can occur in patients with hypokalemic periodic paralysis, which is a rare disorder characterized by recurrent muscle paralysis due to hypokalemia. Hypokalemic periodic paralysis typically is inherited in families, passed down in an autosomal dominant pattern. It can also be acquired in association with thyrotoxicosis. The paralysis occurs when potassium moves intracellularly after a stimulus such as carbohydrate load or exercise. The patient manifests with weakness of extremities, more commonly of the proximal lower extremity muscles (although upper extremities may be affected as well) and hyporeflexia. In between attacks, the neurologic exam is typically normal. The hypokalemia during these attacks can be severe; serum potassium levels may drop as low as 1.5 to 2 mEq/L.33 The exact pathogenesis of the disease is not clear, but in most cases of familial disease (70%), the mutation is in the gene that encodes the dihydropyridine-sensitive calcium channel in skeletal muscle. When the etiology is thyrotoxicosis, the mechanism of intracellular shift is increased sensitivity of Na-K-ATPase to catecholamine. Untreated, potassium tends to return to normal in 6 to 24 hours and paralysis resolves.33
Other etiologies of hypokalemia are a poor oral intake of potassium, or gastrointestinal and urinary losses. Poor oral intake of potassium alone rarely causes hypokalemia, mainly because the kidney has the ability to reduce urinary potassium excretion to 5 to 25 mEq/day if necessary. Usually, another cause of hypokalemia (such as volume depletion) will compound poor oral intake, which will exacerbate hypokalemia.33
Gastrointestinal losses as seen in diarrhea (either infectious, secretory, or osmotic) or vomiting will lead to hypokalemia. Under normal physiologic conditions, only 5 to 10 mEq of potassium are lost in stool. However, acute or chronic diarrhea can cause significant potassium losses in the stool; in severe diarrhea, such as villous adenoma or cholera states, a patient can lose over 100 mEq of potassium per day from the stool. In contrast, vomitus doesn’t contain a lot of potassium (only 5–10 mEq/L). The high hydrogen and chloride losses in the gastric fluid lead to an increased filtered bicarbonate load at the glomerulus. Hence, bicarbonate reabsorption is overwhelmed in the proximal tubule; the result is increased sodium and bicarbonate delivery to the cortical collecting tubule. The volume depletion associated with vomiting activates the renin-angiotensin-aldosterone system and causes aggressive sodium reabsorption at the cortical collecting duct and associated potassium excretion, accompanied by bicarbonate as the nonabsorbable anion.
Urinary losses of potassium are seen in increased mineralocorticoid activity (either from primary or secondary hyperaldosteronism), and from urinary potassium secretion at the cortical collecting duct. The hypokalemia that accompanies diuretic therapy is again primarily due to increased delivery of sodium to the distal nephron where aldosterone activates the electroneutral sodium channel (and resultant potassium secretion by the renal outer medullary potassium [ROMK] channel). See Figure 2-4 and Figure 2-5 for summaries of diuretic effects on the nephron. Please note that although loop diuretics do indeed inhibit the Na-K-2Cl channel of the thick ascending limb, the major mechanism of hypokalemia is the increased delivery of sodium to that aldosterone-sensitive portion of the nephron. The mechanism for hypokalemia for the genetic disorders of Bartter and Gitelman syndromes is identical to the mechanism for diuretic use.33
Clinical manifestations occur with hypokalemia usually at a level less than 3 mEq/L. Weakness usually begins in the lower extremities and spreads to the trunk and upper extremities.32 Severe potassium depletion can lead to muscle cramps, rhabdomyolysis, and myoglobinuria.34,35 A variety of arrhythmias may be seen in patients with hypokalemia. These include premature atrial and ventricular beats, sinus bradycardia, paroxysmal atrial or junctional tachycardia, atrioventricular block, and ventricular tachycardia or fibrillation.33 In addition, electrocardiogram abnormalities often are seen with hypokalemia. The earliest ECG changes associated with hypokalemia are a decrease in the T wave amplitude and ST-segment depression. With more profound hypokalemia (potassium < 3 mEq/L), one can expect T-wave inversions, with PR interval prolongation, widening QRS complex, and an increase in the U-wave amplitude (a U wave is described as a positive deflection after the T wave, best seen in V2 and V3).36 There is certainly variability in the relationship between ECG changes and serum potassium levels; however, 90% of patients with a potassium less than 2.7 mEq/L will manifest some ECG evidence of hypokalemia.33
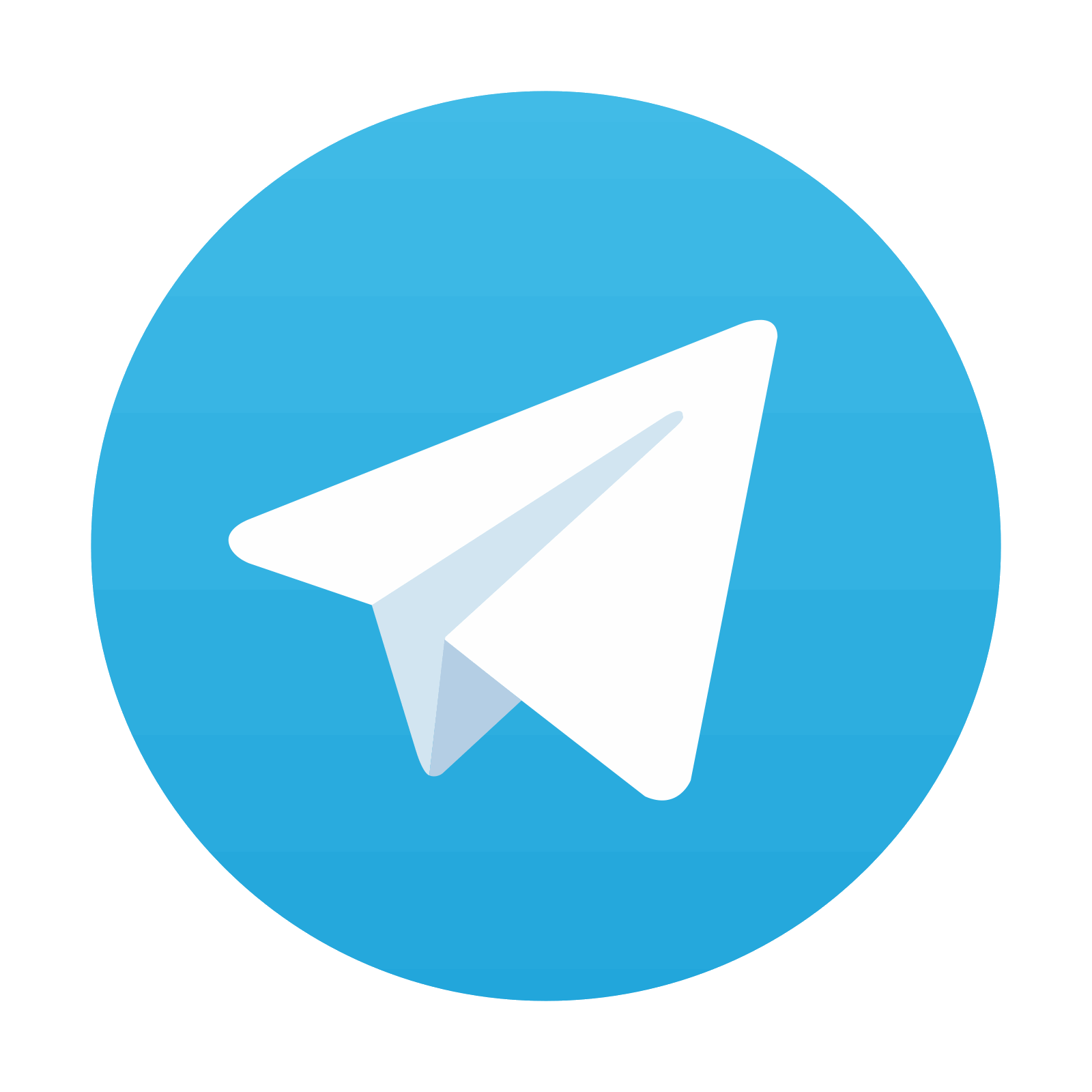
Stay updated, free articles. Join our Telegram channel
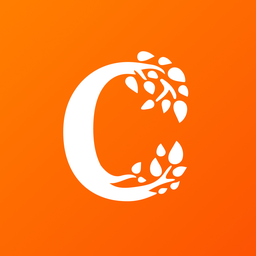
Full access? Get Clinical Tree
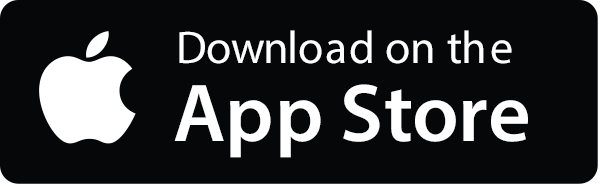
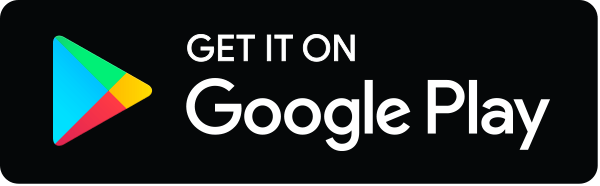