Key Points
- ▪
Anesthesiologists have been key contributors to the field of critical care, both in the advancement of clinical practice and development of technology relevant to the field.
- ▪
Intensive care unit structure, staffing, and utilization varies considerably among developed countries. Studies generally show improved patient outcomes with “high-intensity” staffing models.
- ▪
Low-tidal-volume lung protective ventilation improves mortality for patients with acute respiratory distress syndrome (ARDS). Prone positioning is recommended in patients with severe ARDS.
- ▪
Open lung strategies that include optimization of positive end-expiratory pressure and recruitment maneuvers are of significant research interest and increasingly used in clinical practice. Study results, however, are mixed and do not clearly demonstrate improved outcomes.
- ▪
The Third International Consensus Definitions for Sepsis and Septic Shock (Sepsis-3), a new definition introduced in 2016, emphasizes the key elements of organ dysfunction and dysregulated host response.
- ▪
The Surviving Sepsis Campaign’s guidelines and bundles promote early recognition, administration of antibiotics, and resuscitation with fluids and vasopressors.
- ▪
Hemodynamic resuscitation in shock requires careful evaluation of fluid responsiveness and assessment of end-organ perfusion. There is significant evidence that excess fluid administration can cause harm in critically ill patients.
- ▪
Cancer immunotherapy is a rapidly evolving field in oncology with significant toxicities, including cytokine release syndrome, that present new management challenges for intensivists.
- ▪
Point-of-care ultrasound is increasingly common in critical care practice but more research is needed to evaluate the impact on clinical outcomes.
Acknowledgment
This chapter is a consolidation of two chapters in the 8th edition, Chapter 101 Critical Care Anesthesiology and Chapter 106 Nutrition and Metabolomics. The editors and publisher would like to thank the following authors: Linda Liu, Michael Gropper, and Charles Weissman for their contributions to the prior edition of this work. It has served as the foundation for the current chapter.
Introduction
The history of critical care medicine is relatively brief, dating back to the middle of the previous century. During this time, the field has evolved considerably, with changing approaches to the management of mechanical ventilation, hemodynamic support, sedation, renal replacement therapy, and patient mobilization. Modern critical care is an exciting domain of medicine, with an increasing focus on interprofessional care, family engagement, and long-term outcomes. Outcomes no longer include only attention to survival and length of intensive care unit (ICU) and hospital stay, but also recognition of post-hospital impairments and the new described entity of Post-Intensive Care Syndrome. This chapter begins with a brief overview of the history of critical care medicine, including the specific role of anesthesia intensivists. We will then discuss modern and future ICU structure and management, including focus on resource utilization, staffing and team design, costs, and outcomes. The remainder of the chapter will discuss essential areas of management, including ICU Liberation/A-F Bundle, respiratory failure, shock and hemodynamic support, sepsis, and critical care in oncology.
History of Critical Care Medicine and the Role of Anesthesia Intensivists
Critical care medicine and the development of the first ICU is often credited to Bjorn Ibsen, a Danish anesthesiologist, in 1952. Dr. Ibsen, who was trained in anesthesia at Massachusetts General Hospital, was consulted to help in the care of a 12-year-old girl with poliomyelitis (polio) and respiratory failure. He instituted the approach of manual positive pressure ventilation via a tracheotomy and then applied this to dozens of patients throughout the course of the polio epidemic in Copenhagen. Equally important, Dr. Ibsen implemented the approach of caring for groups of patients with respiratory failure in a dedicated location, thus creating a version of the modern ICU. In addition to this well-cited contribution to modern critical care, the Johns Hopkins neurosurgeon, Walter Dandy, created an earlier version of the modern ICU in 1923, when he opened a three-bed unit for postoperative neurosurgery patients. Max Harry Weil, another key figure in modern critical care, opened a four-bed “shock unit” in 1958 at the Los Angeles County + University of Southern California (LA+USC) Medical Center.
Since the early ICUs, modern critical care has focused on continuous physiologic monitoring and the application of advanced life-sustaining therapies, including mechanical ventilation, extracorporeal membrane oxygenation (ECMO), and continuous renal replacement therapy. One astute observer and practitioner notes that the focus has often been on syndromes—sepsis, acute respiratory distress syndrome (ARDS), acute renal failure, and delirium—rather than specific diseases. The progress in care for these syndromes and other critical care challenges has often been exciting but has also required periodic major shifts in practice, most notably with regard to sedation, mechanical ventilation in ARDS, use of the pulmonary artery catheter (PAC), intensive insulin therapy, and perhaps also goal-directed therapy.
Anesthesiologists, dating back to Dr. Ibsen, have been central to the development of modern critical care, both with the advancement of clinical practice and the development of technology and tools essential to the practice of the specialty. George Gregory, a pediatric anesthesiologist and intensivist at the University of California, San Francisco (UCSF), and colleagues used continuous positive airway pressure (CPAP) in the treatment of neonatal idiopathic respiratory distress syndrome and demonstrated a dramatic improvement in survival. John Severinghaus, who was not an intensivist but certainly a major figure in the field of anesthesiology, was the key contributor to the development of the first blood gas electrode apparatus in the late 1950s. More recently, however, there appears to be a decline in the role of the critical care anesthesiologists in the United States. In 2001, several academic leaders from the United States and Europe authored an editorial raising alarm based on the following: less than 4% of U.S. anesthesiologists have a special certificate in critical care, only 12% of the members of the Society of Critical Care Medicine (SCCM) are anesthesiologists, and relatively few anesthesia critical care diplomates graduate each year from fellowships. By contrast, in Europe, anesthesia intensivists continued to maintain a central role in the field of critical care medicine. In the nearly two decades since this article, it is unclear if much has changed. The number of accredited fellowship programs in anesthesia critical care has declined, and the number of anesthesia critical care diplomates certified by the American Board of Anesthesiology remains low in comparison to other anesthesia subspecialties like pain medicine and pediatric anesthesiology. It is unclear why critical care is a less desirable career path but key factors likely include limited exposure to high quality critical care rotations, lack of faculty mentors, and more favorable financial incentives in anesthesia-based fields. Despite these challenges, the Society of Critical Care Anesthesiologists continues to play a primary advocacy, educational, and mentoring role and memberships have increased over the past several years.
The future roles of anesthesia intensivists in the United States are likely to expand due to the increasing complexity of perioperative medicine, coupled with an aging population in need for critical care. It is also interesting to note a recent proposal by several academic anesthesia leaders from both trauma and critical care of a new paradigm: acute care anesthesiology. Modeled in part on acute care surgery, this new pathway and fellowship would include training in prehospital and emergency care, trauma, and critical care. Specifics of such a training pathway remain unclear but there are compelling reasons to consider this approach as another means of enhancing the anesthesiologist’s role in resuscitation and emergency care.
Intensive Care Unit Structure and Management
Capacity, Utilization, and Cost
ICU bed utilization and cost vary considerably among developed countries. Recognizing that the definition of an ICU bed also varies considerably, the United States, Belgium, and Germany having greater than 20 ICU beds per 100,000 population whereas the United Kingdom, the Netherlands, France, and Spain have fewer than 10 ICU beds per 100,000 population. Similarly, there are also large variations in the volume and type of ICU admissions in developed countries. Part of this can be attributed to differences in bed availability, but it also reflects different approaches to admission and triage that have been well described. A recent large prospective study of noncardiac surgery in Europe demonstrated high mortality rates and significant variability in utilization of critical cares services. Perhaps most striking was the finding that among surgical patients who died, the overwhelming majority (73%) were never admitted to an ICU. Therefore, despite concerns about over-utilization of expensive resources in some countries, this study suggests an alarming under-utilization of essential critical care services.
In the United States, in particular, there is continued growth in ICU beds and cost, whereas occupancy has remained generally flat. In 2005, there were 93,955 ICU beds with occupancy rates of 68%. Critical care costs represented 13.4% of all hospital costs and 0.66% of gross domestic product. By 2010, critical care beds had increased to 103,900 with stable occupancy rates over the previous decade. The greatest growth occurred in neonatal ICU beds with similar, but much lower, growth rates in adult and pediatric ICU beds. Critical care costs also grew to $108 billion, which represented an increase relative to gross domestic product (now 0.72%). The type of growth in ICU beds is also worth noting, as described in a recent analysis of Medicare and Medicaid data. Over 15 years, more than 72% of total ICU bed growth occurred in teaching hospitals. Multivariate analysis also demonstrated that large hospitals with high occupancy and teaching hospitals with high occupancy were most associated with next-year growth in the number of ICU beds. The investigators concluded that this may represent a type of “de facto regionalization,” which may in turn lead to higher quality of care. What is more, they suggest their results also contradict the theory that ICU bed growth and supply represents a type of “demand elasticity,” whereby increasing supply drives demand without clear benefits to patient care and the potential to change practice patterns and increase costs.
Structure and Staffing Patterns in the Intensive Care Unit
Much like the number and use of ICU beds, there is considerable variability throughout the world in the structure and staffing of ICUs. North American ICUs, when compared to the rest of the world, are more likely to have an “open” structure and be stratified into medical and surgical units and are less likely to have 24/7 intensivist presence. ICUs are sometimes described as “open” or “closed;” however, a common research approach is to categorize staffing as “low intensity” (no intensivist or only elective intensivist consult) or “high intensity” (all care directed by intensivist or mandatory intensivist consult). Much attention over the past two decades has focused on the optimal ICU structure and staffing models: specialized versus mixed, “high intensity” versus “low intensity,” optimal physician and nursing staffing ratios, nighttime intensivist staffing, use of advanced practice providers (APPs), and best ICU team structure.
The data do not demonstrate a clear outcomes benefit to specialized units but do show increased mortality for patients “boarding” on a non-primary unit in a specialized system. Many studies, including two large systematic reviews, have investigated outcomes associated with the intensity of ICU staffing. The outcomes have been mixed but generally favor improved mortality and length of stay outcomes with a “high-intensity” model. Data are more limited with regard to provider-to-patient ratios, but a recent retrospective study in the United Kingdom did show a U-shaped relationship between patient-to-intensivist ratios, with an optimal ratio of 7.5. Optimal nursing ratios are also not well described but a large, multinational observational study demonstrated that nurse-to-patient ratios higher than 1.5 are associated with lower risk of in-hospital death. The Working Group on Quality Improvement of the European Society of Intensive Care Medicine (ESICM) recommends 8 to 12 beds as optimal size.
The use of nighttime intensivists is an active area of study, with most studies demonstrating a lack of mortality benefit. It is important to note, however, that there is evidence that nighttime intensivist staffing, in one study, was associated with reduced in-hospital mortality in ICUs with low-intensity daytime staffing. Furthermore, a before-and-after prospective study of 24/7 mandatory intensivist presence showed an association with reduced hospital length of stay and complication rate, as well as improved staff satisfaction and adherence to processes of care. Based on available data, some investigators and critical care organizations (CCOs) have concluded that nighttime intensivists are costly and without significant benefit, whereas others vigorously argue for the value of a 24/7 intensivist model in high complexity, high-volume ICUs, emphasizing that the benefits derive not just from intensivist presence but rather intensivist-led changes in the systematic delivery of care.
Much attention has focused on the best approach to team structure and function in the ICU. As discussed earlier, “open” or “low-intensity” ICUs use hospitalists or emergency medicine physicians to care for patients in the ICU. Many academic and community-based institutions use nurse practitioners (NPs) and physician assistants (PA)—often described as advanced practice providers (APPs)—as core members of the team, largely because of the limited availability of intensivists. Studies demonstrate equivalent mortality and length of stay outcomes when compared to teams that include both residents and pulmonary/critical care fellows. A larger, more recent retrospective study of cohort data from 29 ICUs in 22 hospitals examined the association between exposure to NP/PA ICU staffing and in-hospital mortality. While the patients in NP/PA-staffed ICUs had lower severity of illness and use of mechanical ventilation, the unadjusted and risk-adjusted mortality was similar in ICUs with and without NPs/PAs. In addition to these outcomes, there is evidence of positive perceptions of NPs/PAs from other ICU providers, including physicians, nurses, and respiratory therapists. Accessibility, communications skills, and knowledge of and adherence to guidelines were recognized as core strengths of the NPs and PAs.
In addition to the benefits of nonphysician providers, there is evidence supporting the benefits of multidisciplinary teams and interprofessional practice in the care of critically ill patients. A retrospective study of medical ICU patients in Pennsylvania hospitals demonstrated the greatest mortality benefit to high-intensity intensivist staffing and daily multidisciplinary rounds. A recent review of interprofessional practice describes the robust literature supporting the value of interprofessional practice in the ICU. Pharmacists, respiratory therapists, and physical and occupational therapists are essential providers in the daily care of critically ill patients and improve short-term and longer-term outcomes.
There are guidelines and expectations that address some of the previously discussed aspects of ICU structure. The Leapfrog Group, a major nonprofit “watchdog” organization, rates hospitals on many factors including ICU staffing. The highest Leapfrog rating with regard to the ICU requires all critical care patients to be managed or actively comanaged by a board certified intensivist. The SCCM’s Task Force on Models of Critical Care concludes that an “intensivist-led, high-intensity” team is an “integral” part of care delivery in the ICU. These guidelines do not make a recommendation for either direct management of all patients (i.e., “closed” model) or mandatory consultation by an intensivist. Similarly, the SCCM’s Admission, Discharge, and Triage Task Force makes a 1B recommendation for a high-intensity staffing model with “day-to-day” management either via a fully “closed” structure or with a mandatory intensivist model; the SCCM does not recommend 24-hour intensivist staffing if the ICU operates within a high-intensity structure.
Management and Quality Improvement
The quality of an ICU depends to a significant degree on the management structure, as well as the organizational approach to clinical practice and process, and quality improvement. Management structures vary but have typically included at least a medical director and head nurse or nurse manager as a basic leadership structure. Experts and critical care societies recognize the challenges and importance of selecting and developing skilled critical care leaders. One recent review highlights the need for a leader to maintain and foster “continuity, consistency, and communication” in both clinical practice and the organizational practice. Although this may seem straightforward, the critical care leader often operates in a demanding and complex environment and must have a strong understanding of the broader health system operations and finances. Equally important are strong leadership skills, especially the ability to listen and learn, adapt to changing clinical and organizational circumstances, and constantly serve as a “catalyst for change.”
Beyond development of individual leaders, organizations are increasingly focused on how to best integrate ICUs and their leaders within a critical care structure. The SCCM’s Academic Leaders in Critical Care Medicine Task Force highlights the growing number of academic medical centers now with CCOs. They describe a road map for integration of operations to focus on patient quality, safety, and value: first using a “horizontal” approach with all ICUs operating in a single system with defined responsibility and accountability for value-based care; and then a “vertical” approach using a population health model with care provided across the continuum for all critically ill patients during and after ICU care. The Task Force also presents a follow-up plan for integration of critical care services into a single organization that explicitly includes the academic missions of research, professional development, and education.
High quality clinical practice and appropriate use of expensive and oftentimes limited resources benefit from a clear set of administrative and clinical guidelines. The SCCM recently revised the Admission, Discharge, and Triage Guidelines, which provide useful guidance for institutions developing policies and protocols for patient flow and quality assurance. Experts and professional societies agree that specific protocols of care—applied to practices like central line insertion and management of mechanical ventilation, and broader challenges like the use of the ICU for potentially inappropriate care—can help provide high quality, high value care in a collaborative model. Checklists and bundles often form the foundation for standardization of care and function as a mechanism to implement evidence-based practices and guidelines. The SCCM’s ICU Liberation project, for example, provides the ABCDEF Bundle as a daily, standardized approach to the implementation of the guidelines for the Prevention and Management of Pain, Agitation/Sedation, Delirium, Immobility, and Sleep Disruption in adult ICU patients. Standardization of care is a necessary step, but quality and value also depend on a robust approach to process improvement. Each ICU or CCO must first define the quality and safety metrics to be measured. It is also important that ICUs and CCOs consider the entire scope of quality in critical care. Recent guidelines describing best practices for family-centered care in the ICU highlight the importance of including measures of family support in our definition of quality in critical care. Once defined, the organization’s quality efforts will largely be determined by its ability to collect, analyze, and disseminate data to key leaders and ICU providers and staff.
In summary, critical care is a relatively young medical specialty with a strong history of anesthesiologist involvement since its inception. The practice of critical care medicine continues to grow and evolve rapidly, in terms of medical advancement, practice structure, and integration into the broader landscape of population health. In the next sections we will discuss several of the central disease syndromes and therapeutic modalities that have come to define the modern practice of critical care medicine.
Acute Respiratory Distress Syndrome and Lung Protective Ventilation
Acute Respiratory Distress Syndrome
ARDS is a final common state in acute lung injury characterized by noncardiogenic pulmonary edema, heterogeneous consolidation, decreased lung compliance, and severe hypoxemia. It is a syndrome that involves injury and increased epithelial-endothelial permeability of the alveoli. It can occur as a result of direct chemical injury, systemic inflammation including sepsis, trauma, or numerous other causes common in critically ill patients.
The 2012 Berlin definition of ARDS focused on clarifying the quantification of hypoxia, the acute timing, and the radiographic findings. Using the ratio of arterial oxygen tension to fractional inspired oxygen (PaO 2 /FiO 2 ), ARDS is graded mild if this ratio is 300 mm Hg or less, but greater than 200 mm Hg; moderate if 200 mm Hg or less, but greater than 100 mm Hg; and severe if 100 mm Hg or less. These measurements should be obtained with a positive end expiratory pressure (PEEP) of at least 5 cm H 2 O. The onset must be within 7 days of the presumed inciting insult, and bilateral lung infiltrates must not be fully explainable by other causes including cardiogenic pulmonary edema, effusions, or lobar collapse ( Table 83.1 ).
ARDS Severity | PaO 2 /FiO 2 |
---|---|
Mild | 300-200 mm Hg |
Moderate | 200-100 mm Hg |
Severe | 100 or less mm Hg |
“Baby lung”
Imaging studies have shown that ARDS involves heterogeneous lung consolidation and small regions of remaining aerated lung. As a result, the entire mechanical stress of ventilation is borne by the small remaining regions of aerated lung. This concept has been described as the “baby lung” by some authors, and ventilation strategies have focused on preventing mechanical trauma and optimizing aeration of the remaining functional “baby lung.”
Lung Protective Ventilation
The modern approach to mechanical ventilation in critically ill patients has been characterized by a focus on the prevention of ventilator-induced lung injury. In their landmark ARMA study of tidal volume, the ARDS Network group demonstrated significant decrease in mortality and ventilator days with the use of lung protective ventilation, 6 mL/kg of predicted body weight (PBW) with plateau pressures less than 30 cm H 2 O compared to ventilation at 12 mL/kg of PBW. This lung protective, low-tidal-volume ventilation strategy has become standard of care for patients with ARDS and subsequent studies have associated improved outcomes even in patients without ARDS.
Permissive Hypercapnia
During low-tidal volume ventilation, hypercapnia often occurs. While the avoidance of ventilator-induced lung injury is beneficial, the exact degree and pleomorphic effects of hypercapnia are less clear. There have been mixed data on the effects of hypercapnia on pulmonary inflammation, cellular and immunologic function, and wound healing. Furthermore, hypercapnia may impair right ventricular function, exacerbate pulmonary hypertension, and predispose to cardiac arrhythmias. As a result, most critical care practitioners adopt a pragmatic approach specific to the patients—often described as “permissive hypercapnia”—targeting a pH greater than 7.25. Extracorporeal carbon dioxide removal devices have been in active development to facilitate the management of carbon dioxide clearance during lung injury and low-tidal volume ventilation. However, these invasive devices have yet to prove their clinical utility and effectiveness.
Ventilation Modes in Acute Respiratory Distress Syndrome
In the era of lung-protective ventilation, ventilation strategies have focused on preventing volutrauma, barotrauma, and atelectrauma. Volutrauma is injury to alveoli from excessive volume distention and is closely related to barotrauma from excessive force on the alveolar walls. Atelectrauma is related to the repeated collapse and reopening of alveoli.
Conventional modes of ventilation deliver controlled pressure or flow profiles to the airways at tidal volumes larger than dead space and at rates similar to natural respiration. Gas transport occurs due to cyclic bulk flow into and out of the alveoli. Between the traditional ventilation modes of pressure control and volume control, there has been no significant difference shown in ARDS as long as low tidal volumes are used.
Airway pressure release ventilation (APRV) is a mode of ventilation that holds a high constant inspiratory pressure with periodic brief releases to a lower pressure, with spontaneous respiratory activity superimposed on this pattern. The proposed benefits are increased aeration and reduced cycling of alveolar collapse. A review of observational studies in trauma patients has suggested that early use of APRV may reduce the incidence of ARDS; however, more rigorous studies will be needed to evaluate this hypothesis. Given the risks and unproven clinical benefits of this ventilator mode, its use remains controversial.
High-frequency oscillatory ventilation (HFOV) is another less conventional mode of ventilation that requires a special oscillator pump. HFOV delivers very low volumes, often less than 100 mL, at very high rates, typically hundreds of breaths per minute. In HFOV, because tidal volumes are less than dead space, gas transfer does not depend on bulk flow but on other mechanisms, such as “pendelluft” (swinging air) effect and enhanced diffusion. Whereas initial studies demonstrated improved oxygenation, a subsequent larger clinical trial demonstrated no benefit or increased mortality in patients with ARDS. As a result, HFOV is not recommended, except as a rescue method in refractory hypoxia.
Positive End-Expiratory Pressure and Open Lung Strategies
ARDS is characterized by extensive alveolar collapse. Repeated opening and closing of alveoli during ventilation results in atelectrauma to the lungs. The lung compliance curve is characterized by hysteresis, meaning a higher driving pressure is required to inflate the lungs during inspiration, as compared to lower driving pressure required to keep the lung open during expiration. As a result, the use of PEEP and more generally “open lung” strategies have received significant attention.
Positive End-Expiratory Pressure
PEEP is a continuous pressure held in the airways even on expiration. Increasing PEEP improves oxygenation by increasing functional residual capacity and preventing alveolar collapse on expiration, thus maintaining alveolar recruitment. Excessive PEEP can result in regional or global lung over-distention or exacerbate air trapping. Furthermore, excessive PEEP impairs right ventricular function and venous return, possibly necessitating excess intravenous fluid administration.
Early animal studies demonstrated that the use of PEEP ameliorates ventilation-induced lung injury. As such, a minimum of 5 cm H 2 O of PEEP is recommended by ARDSnet ventilation protocol. However, large clinical studies comparing lower PEEP protocols to higher PEEP protocols failed to demonstrate improvement in clinical outcomes, although oxygenation was improved. The failure of these studies led to the more sophisticated methods of titrating PEEP usually included in open lung strategies (OLSs).
Open Lung Strategies
OLS are the combination of lung protective ventilation with PEEP optimization and possibly recruitment maneuvers. The goal is to maintain aeration of functional lung tissue, thereby preventing atelectrauma, cyclic collapse of the alveoli, and focal lung stress in the remaining compliant regions of the lung. Various PEEP titration strategies have been integrated with recruitment maneuvers into “open lung” strategies.
Esophageal Manometry, Transpulmonary Pressures, and Positive End-Expiratory Pressure
One proposed method is using esophageal pressures to guide determination of the best PEEP. The mechanical stresses of airway pressure can be separated into two components borne by the intrinsic lung compliance and the external chest wall compliance. However, only the lung component, as quantified by the transpulmonary pressure, is involved in ventilation-associated lung injury. The relative contributions of these two components can be difficult to predict and are affected by numerous conditions including ARDS, pulmonary edema, atelectasis, obesity, abdominal compartment syndrome, and burns.
The transpulmonary pressure is calculated by subtracting the pleural pressure, outside the lung but inside the chest wall, from the total airway pressure. Pressure measurements in the esophagus are considered a surrogate of pleural pressure. This can be performed by placing a specifically designed air-filled pressure transducing catheter, similar to an orogastric tube, in the esophagus. With this method, PEEP can be titrated to prevent negative transpulmonary pressures that collapse the lung at the end of expiration. This holds special promise for patients with abnormal chest wall compliance, such as those with morbid obesity.
Titration of PEEP using esophageal pressures in ARDS may allow the use of higher airway pressures without risking lung injury. One clinical study demonstrated improved oxygenation and lung compliance with this approach in association with higher PEEP, but further studies will need to be performed to prove improvement in outcomes of this approach.
Decremental Positive End-Expiratory Pressure Trials
Trials of decremental PEEP have involved raising PEEP to a high level (20-25 cm H 2 O) after a recruitment maneuver. The PEEP was then slowly decreased in a stepwise manner. The final PEEP was set at the level that maximized lung compliance, driving pressure divided by tidal volume. Initial clinical results of OLSs demonstrated promising physiologic improvement in patients with severe ARDS. However, these initial studies were heterogeneous in nature, and in the largest randomized prospective trial of an open lung protocol, 6-month mortality, ventilator days, and risk of pneumothorax was actually increased. Criticisms of this latter study include use of high recruitment pressures resulting in increased barotrauma and hemodynamic instability. Further research will need to be performed to elucidate the best recruitment and PEEP strategies in ARDS.
Recruitment Maneuvers
The goal of recruitment maneuvers is to aerate previously collapsed lung, thereby improving gas exchange and overall lung stress. The most common recruitment maneuver is simply holding sustained airway pressure for a set time period, typically 30 to 40 cm H 2 O for 30 to 40 seconds. This therapy is not benign, and common complications include transient hemodynamic instability, oxygen desaturation, ventilator dyssynchrony, or rarely pneumothorax. As such, the patient should be closely monitored during and after recruitment manuvers. Another potentially gentler method of recruitment is incremental PEEP titration. PEEP is increased in a stepwise manner of 2 to 5 cm H 2 O every 3 to 5 minutes until there is a worsening of respiratory or hemodynamic parameters. However, there is little evidence of the superiority of any specific recruitment maneuvers. Furthermore, there is an overall lack of strong evidence for improved clinical outcome; thus, the role of recruitment maneuvers in ventilation strategies is uncertain.
Prone Positioning
Prone positioning has a number of physiologic benefits in ARDS. Ventilation-perfusion matching and lung aeration is improved, and parenchymal heterogeneity is reduced. This likely contributes to a protective effect against ventilator-induced lung injury. In the largest study of only patients with severe ARDS, defined as PaO 2 :FiO 2 less than 150 mm Hg, 28-day mortality was significantly reduced. As such, prone positioning is recommended in severe ARDS, with the caveat that benefits were demonstrated at centers with deep experience. At centers with less experienced practitioners, prone positioning may be technically challenging with risk for increased serious complications.
Self-Induced Lung Injury
In negative pressure spontaneous respiration, the chest wall exerts a negative pressure on the lungs. In instances of increased respiratory drive and lung injury, this can induce lung injury without positive pressure ventilation. In animal studies, chemically induced hyperventilation alone has been demonstrated to induce lung injury. In mild lung injury, spontaneous respiration is associated with better oxygenation, improved dependent lung aeration, and decreased diaphragm atrophy. However, in severe lung injury, spontaneous respiration can be associated with increased lung injury.
Neuromuscular Blockade
Neuromuscular blocking agents have been used to facilitate synchrony with mechanical ventilation by removing spontaneous chest wall and diaphragm motion. This lowers ventilation pressures, decreases risk of barotrauma, and improves oxygenation. Additionally, some animal data suggest the neuromuscular blocking agent, cisatracurium, has direct lung-protective properties through antiinflammatory actions. One observational study comparing vecuronium and cisatracurium found significantly fewer days on the ventilator with cisatracurium, although no difference in mortality or other clinical outcomes. In a prospective study of neuromuscular blockade in ARDS, the ACURASYS study, cisatracurium administration for the first 48 hours was found to decrease lung injury and mortality in patients with severe ARDS whose PaO 2 /FiO 2 was less than 150 mm Hg. Additionally, no increased incidence of weakness or critical illness myopathy was seen with this short duration of use. However, this study was criticized for being relatively small, single centered, and for having insufficiently long follow up to evaluate critical illness myopathy. To address these concerns, the larger, multicentered Reevaluation Of Systemic Early Neuromuscular Blockade (ROSE) study was performed by the Prevention and Early Treatment of Acute Lung injury (PETAL) Network investigators. In this more recent trial, early continuous neuromuscular blockade did not lower all-cause mortality at 90-days. There were no consistent between-group differences in outcomes at 3, 6, and 12 months. However, when compared to the ACURASYS trial, patients in the ROSE trial received higher levels of PEEP, and those not paralyzed also received lighter sedation, potentially contributing to the difference in observed outcomes.
Noninvasive Positive-Pressure Ventilation and High-Flow Nasal Cannula
CPAP and bilevel positive airway pressure (Bilevel PAP) masks have been shown to be effective first-line treatment in respiratory failure due to acute cardiogenic pulmonary edema and chronic obstructive pulmonary disease (COPD). However, their use in ARDS is controversial. Although avoidance of endotracheal intubation could have many benefits, studies have failed to show benefit in the need for intubation or mortality. In fact, in one study, noninvasive positive-pressure ventilation (NPPV) was associated with increased mortality compared to high-flow nasal cannula (HFNC). Patients better tolerate HFNC than mask NPPV, and HFNC was not found to be inferior in intubation rates or mortality compared to NPPV. However, the HIGH trial, a prospective, multicenter, randomized study in immunocompromised patients, failed to show any benefit in mortality, intubation rates, or even patient comfort. Further research in the role of HFNC and other nonmask forms of NPPV is needed.
Summary of Evidence-Based Ventilation in ARDS and Future Research
The use of lung protective ventilation with 6 mL/kg of PDW is highly recommended. The use of PEEP at a minimum of 5 cm H 2 O and titration based upon the ARDSnet protocol is recommended, but the optimal PEEP level is unclear. In severe ARDS, the early use of prone positioning has been shown to improve mortality. HFOV is not recommended as a primary ventilation method. Future research will need to establish the role of recruitment maneuvers and optimal PEEP titration strategies. The role of noninvasive ventilation, neuromuscular blockade, and the risk of self-induced lung injury will also need to be clarified in the treatment of ARDS.
Ultra low-tidal volume ventilation, 4 mL/kg of PDW or less, continues to be investigated. The technology of artificial gas exchange devices, extracorporeal carbon dioxide exchangers, or venovenous ECMO support, continues to be advanced. Finally, despite negative trials of aspirin, statins, and beta-agonists, new pharmacologic and biologic therapies including mesenchymal stem cell therapies continue to be investigated.
Management of Pain and Agitation
Sedatives are some of the most commonly administered medications in the ICU. The purpose of sedatives is to manage agitation to ensure the safety of the patient and care providers, and to prevent dangerous events such as unintended extubation. The first step to effectively manage agitation is to identify and address underlying causes. Pain is a common cause of agitation in the critically ill and is often underappreciated. Delirium is extremely common in the critically ill and is associated with adverse outcomes including increased length of stay and mortality. Delirium can manifest as hypoactivity or with agitation. The first-line interventions should be nonpharmacologic.
When sedatives are needed, targeting light sedation is recommended. The use of light sedation is not associated with increased posttraumatic stress disorder, and may be associated with shorter ventilator time or even decreased mortality. The use of daily sedation interruptions or nursing-driven protocols with standardized tools are both effective methods to achieve this goal. Two well-validated scales for sedation assessment are the Richmond Agitation-Sedation Scale and the Sedation-Agitation Scale. Some even propose protocols that try to completely avoid traditional sedatives and focus primarily on analgesia and control of agitated delirium with haloperidol. Though this approach may be associated with shorter length of stay and mechanical ventilation, further study is needed to confirm this finding. Furthermore, there is a suggestion that benzodiazepines lead to increased hyperalgesia with administered opioids. This should be considered in patients who require opioids for pain.
Choice of specific sedative agent is controversial. However, benzodiazepines, when compared to propofol or dexmedetomidine, may be associated with increased delirium and length of mechanical ventilation. Propofol, with the benefits of short duration and rapid titration, is a very common choice, but may cause hypertriglyceridemia or, rarely, propofol infusion syndrome. Propofol infusion syndrome is a serious complication associated with high dose and prolonged infusion duration that presents with metabolic acidosis, electrocardiogram (ECG) and conduction abnormalities, and rhabdomyolysis. Dexmedetomidine is increasingly being used in the ICU. Several studies have suggested that it is less deliriogenic than other sedatives, or may even have prophylactic benefits against delirium.
Neuroleptic drugs such as haloperidol are rarely used as primary sedatives, but can be used to control agitated delirium that may result in harm to patient or staff. However, studies have not shown clear benefit with the use of antipsychotics in the prevention or treatment of delirium. Furthermore, many patients who start treatment with antipsychotics in the ICU are discharged with a prescription for antipsychotics with the accompanying dangerous side effects associated with long-term use.
Pragmatically, regardless of the specific sedative agent or protocol and scales used, the evidence points toward using a systematic and adaptive practice of minimizing psychoactive agents, while maintaining safety of the patient and care providers.
Sepsis and Shock
Infection, Systemic Inflammatory Response Syndrome, and Sepsis
Sepsis is one of the most common diagnoses treated in ICUs. It is estimated that at least 1.7 million patients develop sepsis every year in the United States alone, the majority of whom are over the age of 65. Mortality, which reaches 10% in patients with sepsis and 40% in patients with septic shock, is typically a result of multiple organ dysfunction synrome (MODS). Sepsis has been defined as a “life-threatening organ dysfunction that is a result of dysregulated host response to infection.”
The most recent definition of sepsis (“Sepsis-3”), introduced in 2016 by the SCCM/ESICM task force, emphasizes the importance of organ dysfunction for sepsis diagnosis, as opposed to systemic inflammatory response syndrome (SIRS). Although features of SIRS (tachycardia, tachypnea, fever/hypothermia, leukocytosis/leukopenia) are found in many patients with sepsis, SIRS is not a specific response to infection and can develop in many noninfectious conditions (major trauma, major surgery, burns, necrotizing pancreatitis). All patients with sepsis, however, have clinical or laboratory evidence of acute organ dysfunction (e.g., hypotension, hypoxemia, altered mental status, oliguria).
The transition from infection to sepsis is believed to occur when the inflammatory response to the pathogen becomes dysregulated, spreading beyond the original site of infection and leading to systemic organ dysfunction. The organ dysfunction has been defined as an increase in the Sequential Organ Failure Assessment (SOFA) score by at least 2 points. The SOFA score uses a simple numeric assessment (0-4) of neurologic, cardiovascular, respiratory, renal, liver, and hematologic function to assess the severity of a patient’s physiologic deterioration ( Table 83.2 ). While SOFA score per se is not diagnostic of sepsis, the increase in SOFA score in a patient with a suspected or confirmed infection indicates a substantially increased risk of in-hospital mortality. Clinicians must maintain a high suspicion for infection in all patients that manifest worsening organ function. It is important to note, however, that in approximately half of the cases of sepsis, the causative microbe is never identified. This is because of the limitations of currently used culture-based microbiology methods, inadequate sampling, or concurrent treatment with antimicrobials. Because the initial clinical presentation of sepsis is variable and laboratory findings are nonspecific, detecting sepsis early remains a clinical challenge.
System | Score0 | 1 | 2 | 3 | 4 |
---|---|---|---|---|---|
Central nervous system
| 15 | 13-14 | 10-12 | 6-9 | <6 |
Respiration
| ≥400 | <400 | <300 | <200 with respiratory support | <100 with respiratory support |
Cardiovascular | MAP ≥70 mm Hg | MAP <70 mm Hg | dopamine <5 or dobutamine (any dose) ∗ | dopamine 5.1-15 or epinephrine ≤0.1 or norepinephrine ≤0.1 ∗ | dopamine >15 or epinephrine>0.1 or norepinephrine >0.1 ∗ |
Liver
| <1.2 | 1.2-1.9 | 2.0-5.9 | 6.0-11.9 | >12 |
Coagulation
| ≥150 | <150 | <100 | <50 | <20 |
Renal
| <1.2 | 1.2-1.9 | 2.0-3.4 | 3.5-4.9 <500 | >5.0 <200 |
∗ Catecholamine doses are given as μg/kg/min for at least 1 hour.
Septic Shock
In patients with septic shock, the dysregulated host response to infection is associated with severe circulatory, cellular, and metabolic abnormalities. Required for the clinical diagnosis of septic shock is elevation of plasma lactate levels greater than 2 mmol/L and the requirement for vasopressor support to maintain mean arterial pressure (MAP) greater than 65 mm Hg despite adequate fluid resuscitation. Circulatory failure that occurs in septic shock is a result of vasodilation and increased vascular permeability, which lead to decreased effective circulating volume. Myocardial dysfunction develops in a significant percentage of patients with septic shock. Tissue perfusion is further impaired by tissue edema and aberrant activation of coagulation at the microvascular level. The ability of tissues to extract and utilize oxygen is also impaired, likely due to sepsis-induced mitochondrial dysfunction. Reflecting these perfusion and cellular abnormalities are supranormal values of oxyhemoglobin saturation in mixed venous or central venous blood often found in patients with septic shock.
Surviving Sepsis Campaign
The high prevalence, high mortality, and mounting costs of intensive care have led to continuous worldwide efforts to identify the best practices in sepsis, resulting in the Surviving Sepsis Campaign. These recommendations were published first in 2004 and revised in several subsequent editions. They emphasized sepsis as a medical emergency and advocated for management to be initiated immediately upon recognition.
It is broadly accepted that an early administration of appropriate antibiotic therapy, within 1 hour of sepsis recognition, is a key intervention to decrease patient mortality. Additional source control (i.e., drainage of an abscess or resection of infected tissues) may be critical in situations in which antibiotic therapy alone would not be curative (e.g., necrotizing soft-tissue infection).
The remaining recommendations for the management of sepsis and septic shock focus on physiologic stabilization (restoration of perfusion, adequate pulmonary gas exchange while avoiding ventilator-induced lung injury, correction of electrolyte abnormalities, and more). Administration of intravenous fluids (30 mL/kg or individualized) is recommended in all infected patients who demonstrate clinical or laboratory signs suggestive of impaired tissue perfusion (i.e., hypotension or lactate elevation greater than 4 mmol/L). Treatment with vasopressors is typically initiated when hemodynamic stability cannot be restored with fluid administration alone. To that end, norepinephrine is the recommended vasopressor of choice. Corticosteroids are helpful in decreasing vasopressor requirements and shock reversal but their impact on mortality is less clear.
Despite the best available supportive care, shock develops in a subset of patients with sepsis that is refractory to treatment with antibiotics, fluids, vasopressors, and corticosteroids, and ultimately progresses to the failure of most organ systems. This is described as MODS. Encephalopathy, ARDS, liver failure, renal failure, and coagulopathy characterize this clinical picture that has an extremely high mortality.
Hemodynamic Support in Septic Shock
Over the last 20 years, it has been intensely studied whether specific hemodynamic or metabolic indices should be uniformly achieved during resuscitation, with proponents of such an approach introducing the term “goal-directed therapy.” Under such management, predefined “best” values of central venous pressure (CVP), MAP, central venous oxygen saturation (ScvO 2 ), or urine output would be achieved through administration of crystalloids, blood, vasopressors, and inotropes. While this protocolized approach was shown to decrease mortality in a single-center study by Rivers in 2001, more recent multicenter clinical trials (ProCESS, ARISE, and ProMISe) have questioned the benefit of a stringent protocolized resuscitation. Likely due to the improvements in general critical care over the last decade, these studies failed to demonstrate the survival benefit of protocolized hemodynamic resuscitation when compared to standard care. Hemodynamic resuscitation in shock is discussed in further detail in the following section.
Hemodynamic Resuscitation in Shock
Circulatory shock is a common condition in intensive care. It is defined by insufficient end-organ perfusion and, if left untreated, leads to end-organ dysfunction and failure. The purpose of fluid administration in circulatory shock is to increase cardiac output (CO) and thus increase end-organ perfusion. Traditional fluid resuscitation strategies were based on empiric intravenous fluid administration and static markers of cardiac preload such as CVP or pulmonary capillary wedge pressure.
In the landmark 2001 paper, Rivers and associates demonstrated a significant mortality decrease, 30.5% versus 46.5%, in patients with septic shock with the use of an early goal-directed therapy (EGDT) protocol versus standard care. EGDT was an emergency department-based protocol that focused on meeting concrete targets of CVP, oxygen delivery, and ScvO 2 . This involved aggressive fluid resuscitation, allogenic red blood cell transfusion, and inotropic support per algorithm. Subsequent studies called various parts of this aggressive protocol into question. Three larger, multicenter prospective randomized studies showed no difference between EGDT and standard care. Thus, the benefit of the EGDT may have been due to the early intervention by a specialized treatment team, rather than the aggressive therapy protocol.
This data emerged with increasing evidence of harm caused by excessive intravenous fluid administration. One multicentered retrospective study of patients in septic shock demonstrated an association between positive fluid balance, higher CVP, and mortality. Another multicentered observational study associated positive fluid balance with increased mortality and renal failure. In a prospective randomized trial, a conservative fluid protocol demonstrated significantly shorter ICU length of stay and fewer ventilator days in patients with ARDS, but no difference in mortality. Data from the CLASSIC trial demonstrated feasibility of a fluid restrictive protocol in critically ill septic patients without apparent worsened outcomes, but it was underpowered for many important clinical outcomes.
Although fluid resuscitation in septic shock is standard of care, there is no high-quality large-scale clinical outcomes data on the efficacy of intravenous fluid resuscitation. In the thought-provoking multicentered 2011 FEAST trial, children presenting with septic shock in a resource-poor setting were prospectively randomized to intravenous fluid administration. Surprisingly, mortality was increased in the fluid administration arm, primarily from cardiovascular collapse. In adults, a single center randomized study of patients with septic shock in a resource-limited setting also saw increased mortality when randomized to more aggressive intravenous fluid administration. Given the specific resource-limited setting and patient population in these two studies, the results are not generalizable. However, these unexpected results do challenge the traditional dogma of intravenous fluid administration in septic shock and raises questions about the Surviving Sepsis Campaign’s recommendation for 30 mL/kg crystalloid bolus in the initial management of septic shock.
Modern fluid resuscitation strategies in circulatory shock are shifting away from empiric intravenous fluid volumes toward a rational approach of supporting perfusion pressure, identifying end-organ malperfusion, evaluating fluid responsiveness, and administration of fluids to augment CO in a targeted manner.
End-Organ Perfusion
Since the goal of fluid resuscitation is to restore end-organ perfusion, the first step should be to identify end-organ malperfusion. There are many surrogates used as indicators for end-organ perfusions, but they all have their limitations and confounders. The most commonly used markers in shock are urine output, lactate, and base deficit.
Urine Output
Urine output is almost universally measured in critically ill patients. Urine output of less than 0.5 mL/kg/hour is a common cut-off used in adults as a marker for systemic malperfusion or hypovolemia. However, urine output is not directly correlated to end-organ perfusion and injury. There are many confounders, especially in the critical care setting, including neurohumoral effects, diuretic use, intrinsic kidney injury, obstructive uropathies, and exogenous vasopressin administration. Despite these many limitations, the ubiquity of this measurement has made it a core component of evaluating a patient in shock. There is further research examining real-time urine output rate as a more effective monitor in shock and may be associated with decreased rates of acute kidney injury.
Lactate
Lactate is one of the most commonly measured markers of organ ischemia. Lactate is a product of glycolysis and even under normal physiologic conditions is produced at a high rate. Although production may be increased by tissue-level hypoxia, there are many other causes of increased lactate production that are not secondary to lack of perfusion. These include β-adrenergic activation, acute lung injury, or medications. Further, lactate clearance can be reduced by hepatic or mitochondrial dysfunction. Thus, although elevated lactate levels are associated with poor outcomes in shock states, it may not be a specific marker for organ malperfusion or ischemia.
Standard Base Deficit
Standard base deficit is a calculated value based on blood gas measurement as a gauge of metabolic acidosis. It approximates the amount of base that must be added to in vitro blood at 37°C and P CO2 of 40 mm Hg to bring the pH to 7.4. Like lactate, elevated base deficit has been associated with mortality and poor clinical outcomes in shock states, especially in trauma. However, base deficit is affected by many factors. It can also be confounded by nonperfusion-related conditions including sodium and chloride concentrations, hypoalbuminemia, respiration, exogenous sodium bicarbonate, or sodium chloride administration.
Other Tissue-Specific Perfusion Markers
The three traditional markers of end-organ perfusion discussed thus far are global markers that may be normal even when there is regional organ level malperfusion. Newer techniques, such as near-infrared spectroscopy, transcutaneous oximetry, and gastric pH/tonometry, may provide a more specific and sensitive endpoint target in shock. Currently, however, these technologies are primarily used in research and there is no strong clinical evidence of superior clinical outcomes compared to traditional endpoints. Future developments will also likely involve organ or inflammation-specific biomarkers.
Cardiac Output
The purpose of intravenous fluids during resuscitation is to increase CO; therefore, it is important to evaluate the adequacy and the response of CO to avoid excess intravenous fluid administration. There have been an increasing number of noninvasive technologies available to measure CO in the ICU to supplement the physical examination. Each has its own strengths and weaknesses, but no single technology has been proven clinically superior. The absolute measurement accuracy of many of these devices is highly variable, especially under rapidly changing hemodynamic conditions. Although the different methods have been validated individually, actual interdevice agreement is likely poor. Measurements from one device cannot be directly compared to another; this may be true even within the same technology from different manufacturers. Several of the most commonly used CO measurement techniques are reviewed in the next section. Refer to Chapter 36 on cardiovascular monitoring.
Pulmonary Artery Catheter, Thermal Dilution
PACs are considered the gold standard of CO measurements. In addition to direct measurement of pulmonary artery and pulmonary capillary wedge pressures, it allows for thermal dilution measurements of CO. This method involves injecting a known amount of cold solution into the right atrium and measuring the temperature change over time in the pulmonary artery. Based on the Steward-Hamilton equation, the area under the curve of the temperature change is inversely proportional to the CO. Current PACs also offer continuous CO measurements with thermal heating elements proximal to the pulmonary artery. Although thermal dilution PAC is the gold standard, precision error is approximately 15% to 20% even in idealized laboratory conditions. This precision is further worsened in patients with low CO or severe tricuspid regurgitation. Although PACs were once widely used in critically ill patients, their routine use has fallen out of favor after multiple studies failed to demonstrate improved clinical outcomes and the possibility of increased complication rates with PAC usage. In select patients, PAC still provides valuable information during shock resuscitation and can be used to monitor pulmonary artery pressures, CO, and CO responses to intravenous fluid challenges.
Transpulmonary Thermal Dilution or Lithium Dye Dilution
Transpulmonary thermal or other dye dilution techniques use the same principles of the Steward-Hamilton equation; however, the indicator solution is injected into the superior vena cava and the change is measured near the aorta, typically on a femoral or axillary arterial line. The advantage of this technique is that it does not require placement of a PAC and is easy to use. Accuracy and precision are similar to PAC measurements, although it also becomes inaccurate at low CO. These systems also allow the measurement of other physiologic parameters such as extravascular lung water.
Pulse Contour Analysis
Pulse contour analysis measures CO by analyzing the morphology of the arterial pressure waveform and thus requires a good quality arterial line tracing. By measuring the area under the systolic portion of the curve and estimating the arterial impedance, the stroke volume can be derived. In modern systems, this is combined with proprietary algorithms with more advanced waveform analysis. These methods suffer from drift and inaccuracy in rapidly changing hemodynamic states, so they are often coupled with thermal or dye dilution techniques for intermittent calibration. The advantages of these devices are in providing a continuous measurement of stroke volume and stroke volume variability, while avoiding the need for central line. These devices have been investigated in perioperative hemodynamic optimization with mixed results in improving clinical outcomes.
Electrical Bioreactance and Impedance
Bioreactance and bioimpedance methods measure CO by measuring the changing properties of an electric field placed across the thorax. This is related to the movement of blood into and out of the chest and thus the CO. The main advantage of this technique is that it is minimally invasive and only requires several electrodes placed on the chest. The accuracy of these devices can be variable depending on the clinical situation, and measurements may correlate poorly with other techniques.
Transthoracic and Transesophageal Echocardiography
Focused cardiac ultrasound and echocardiography can be used to measure stroke volume and stroke distance. This is performed by pulse wave Doppler integration of systolic flow at the left ventricular outflow tract (LVOT). This is typically performed from the transthoracic apical window or the deep transgastric esophageal window. The volume-time integral (VTI) of the flow velocity curve gives the stroke distance. The VTI multiplied by the LVOT cross-sectional area gives the stroke volume, but VTI alone can be used as an index of stroke volume. However, this technique is very operator dependent, can be labor intensive, and does not account for aortic valve regurgitation. Furthermore, some studies have reported poor agreement with other methods of CO measurement.
Esophageal Doppler operates in a similar manner, except the probe is placed in the esophagus and measures velocities in the descending aorta. These velocities are then indexed to the patient to estimate the total CO.
Mixed Venous and Central Venous Saturation
Mixed venous oxygen saturation drawn from the pulmonary artery is a powerful indicator of the balance between global oxygen delivery and global oxygen consumption. Although CO can be calculated using Fick’s principle with assumptions about oxygen consumption, a low mixed venous oxygen saturation, less than 65% to 70%, indicates a deficit of oxygen delivery relative to oxygen consumption. Since it is a global measure, organ-specific ischemia may be present even with normal or elevated mixed venous saturations.
Measurement of mixed venous oxygen saturations requires placement of a PAC. As a result, some have proposed central venous saturation from the vena cava as a surrogate. Unfortunately, the relationship between central venous saturation and mixed venous saturation has been found to be variable.
Fluid Responsiveness
The hemodynamic purpose of intravenous fluid administration is to increase global oxygen delivery by increasing CO. In this regard, the administration of intravenous fluid is only beneficial if it increases CO. A patient is considered “fluid responsive” if they are able to increase their CO to a fluid bolus.
Traditional static indicators for fluid administration were measurements of filling pressures to the left or right ventricle. These included CVP and pulmonary capillary wedge pressures. These measurements also have their ultrasound corollaries: inferior vena cava diameter, end diastolic, and end-systolic ventricular diameter. Unfortunately, despite their widespread use, these static indicators of preload, and even their trend, have been found to be poor predictors of volume responsiveness.
As a result, dynamic indicators of fluid responsiveness have become increasingly used. The most straightforward dynamic test of fluid responsiveness is a fluid challenge with measurement of CO before and after. Typically, a small bolus of approximately 100 to 250 mL of intravenous fluid is used. Similarly, a passive leg raise test resulting in transient autotransfusions from the lower extremities has also been shown to be an effective predictor of fluid responsiveness.
Other dynamic indicators depend upon cardiopulmonary interactions and the consistent variations of intrathoracic pressure created by controlled mechanical ventilation to create detectable beat-to-beat variations in stroke volumes. As positive pressure breath is delivered, right ventricular preload is decreased and afterload increased. Meanwhile, the left ventricle preload experiences a transient increase and then a decrease as left ventricular afterload increases. This process is reversed on expiration. If the stroke volume is sensitive to these swings in preload, the stroke volume will vary significantly during the respiratory cycle and is indicative that the patient is likely volume responsive. The measured parameters can be stroke volume variation, pulse pressure variation, or inferior vena cava distensibility on ultrasound; various studies have reported different cut-offs for predicting volume responsiveness. These techniques may not be accurate in arrhythmias, right or left heart failure, or low-tidal volume ventilation. However, these dynamic indicators have been shown consistently superior in predicting volume responsiveness compared to static preload measures.
Summary of Fluid Resuscitation in Shock
The goal of intravenous fluid administration in shock is to restore end-organ perfusion by augmenting CO. There is increasing evidence that excess intravenous fluid administration is harmful in critically ill patients. Thus, the patient should be assessed for end-organ malperfusion, insufficient CO, and likelihood of fluid responsiveness prior to and during fluid resuscitation.
Care for Patients Receiving Cancer Immunotherapy in the Intensive Care Unit
Cancer immunotherapy is a rapidly evolving field of oncology. Two major therapeutic approaches utilized in cancer immunotherapy are based on either enhancing the patient’s own antitumor immunity (immune checkpoint inhibitors, cytokines, vaccines) or administration of tumor-reactive immune cells (chimeric antigen receptor [CAR] T cells, T-cell receptor engineered T cells). The outcomes of these therapies are currently studied in several hundreds of clinical trials. Remarkable results have already been achieved in certain types of cancer (melanoma, leukemias, lymphomas) for which immunotherapy is now being used as a standard of care.
Besides the desired antitumor effects, immunotherapy can also trigger unique toxicities resulting from excessive immune system activation. These may be restricted to certain organs (colitis, pneumonitis) or be a systemic inflammatory response (cytokine release syndrome [CRS]). When severe, immunotherapy toxicities can be life-threatening and their management may require admission to the ICU for continuous monitoring and supportive care. Intensivists thus increasingly find themselves leading and coordinating multidisciplinary care for complex oncologic patients.
Immune Checkpoint Inhibitors
Immune checkpoint inhibitors are the most commonly administered form of cancer immunotherapy. These agents enhance antitumor activity of the patient’s own T cells by blocking certain T-cell inhibitory signals. FDA-approved inhibitors of PD-1 (pembrolizumab, nivolumab), PD-L1 (atezolizumab, avelumab, durvalumab), and CTLA-4 (ipilimumab) are used in treatments of various solid tumors including melanoma, non-small-cell lung cancer, head and neck squamous cancers, and renal cell carcinoma. These agents can produce a unique set of side effects termed immune-related adverse events, which may be dermatologic, gastrointestinal, hepatic, endocrine, pulmonary, cardiac, or neurologic inflammatory complications. These side effects typically manifest weeks to months after initiation of the therapy.
The mainstay of the management in cases of moderate- and high-grade toxicity is the interruption of the checkpoint inhibitor and administration of corticosteroids. Tumor necrosis factor-α antagonists have been used in cases refractory to steroids. ICU monitoring and supportive care may be required in cases of severe pneumonitis (oxygen therapy, mechanical ventilation), myocarditis (inotropic support, antiarrhythmics), severe enterocolitis (fluid and electrolyte repletion), liver failure, and adrenal insufficiency. Echocardiography should be performed in patients with new onset dyspnea, pulmonary edema, or hypotension. Patients receiving prolonged immunosuppression for the treatment of checkpoint inhibitor toxicity are at an especially high risk of infectious complications (opportunistic infections, sepsis).
Chimeric Antigen Receptor T Cells
CAR T cells are genetically engineered T cells that have the ability to bind to target tumor cells, undergo supraphysiologic activation, and cause tumor cell lysis. Upon completion of an ex vivo manufacturing process, CAR T cells are infused into patients and patients typically remain monitored for several days in the hospital setting. Currently, CAR T cells are used in the treatment of certain relapsed/refractory hematologic malignancies (leukemias, lymphomas, multiple myeloma) but clinical trials in patients with solid tumors are also ongoing. Two CD19-directed CAR T-cell products for treatment of B-cell malignancies (non-Hodgkin lymphoma, acute lymphoblastic leukemia) have been FDA approved and cases of complete disease remission have been reported.
However, CAR T-cell therapy is frequently associated with toxicities that can range from minor (fatigue, fever, myalgias) to life-threatening (shock, multiple organ dysfunction). These toxicities are primarily driven by proinflammatory cytokines (IL-6, IFNγ) that are released upon CAR T cell–tumor cell interaction. Of importance to ICU clinicians are severe forms of CRS and neurotoxicity, which require ICU monitoring and management. CRS typically manifests within several days after CAR T-cell infusion by a spectrum of fever, tachycardia, hypotension, or respiratory insufficiency. The onset of neurotoxicity (encephalopathy, aphasia, or seizures) can be delayed and is not necessarily preceded by clinically significant CRS. Fatal cases of diffuse cerebral edema have been reported.
The mainstay of the therapy of severe forms of CRS is the administration of antiinflammatory agents (IL-6 antagonists, corticosteroids) in conjunction with individualized supportive care (fluid resuscitation, vasopressors, mechanical ventilation, renal replacement therapy). Neurotoxicity is treated with anticonvulsants and corticosteroids. It is crucial that other precipitating factors of neurologic, hemodynamic, or respiratory decline that are common in patients with advanced cancer are always actively excluded. Sepsis, which often resembles CRS, is a frequent cause of morbidity and mortality in this patient population.
Point-of-Care Ultrasound in Critical Care
As a result of improving technology, decreasing cost, and widening availability, the use of point-of-care ultrasound (POCUS) has taken an increasing role in the practice of critical care. The uses of ultrasound in the critically ill are manifold, from vascular access to cardiopulmonary evaluation. POCUS allows for rapid and repeated assessments of the critically ill patient at the bedside to augment traditional physical examination and physiologic monitors. Because techniques and technologies continue to evolve, there are a multitude of published protocols. Although the exact protocol used is of less significance, the specific skills and applications of ultrasound will likely become a core competency in the ICU. Ultimately, ultrasound is another tool in the armamentarium of the intensivist that requires understanding of physiology and expert clinical decision making for safe and beneficial use.
Vascular Ultrasound
Vascular cannulation is a vital skill in the ICU. Ultrasound guidance can be used before the procedure to confirm anatomy and vessel patency or, preferentially, it can be used in real time during the procedure. There are different techniques, the two most common being out-of-plane needle insertion with short axis view of the vessel, or in-plane needle insertion with a long axis view of the vessel. Ultrasound can be used for cannulating central venous structures, arteries, or even peripheral veins.
Central venous access is one of the most common procedures in the ICU. The use of ultrasound imaging to assist catheter insertion is a Grade 1B recommendation by the SCCM guidelines. For the cannulation of the internal jugular or femoral veins, SCCM offers an even stronger Grade 1A recommendation. For other central venous cannulation sites, such as the subclavian or axillary, ultrasound guidance may still improve success and reduce complications, but the evidence is not as definitive. Specific recommendations are given for the use of real-time imaging in the short axis when using ultrasound; however, the conclusions are weaker. Although the long-axis ultrasound view of vessels can have benefits such as reducing posterior wall puncture, the short-axis orientation affords a view of surrounding structures, requires less training, and has been shown to have a higher success rate in some studies.
Arterial cannulation is another extremely common procedure in the ICU and the use of ultrasound guidance is a Grade 2B recommendation. A recent meta-analysis of randomized control trials concluded real-time ultrasound guidance decreased time to cannulation and hematoma formation. A study of radial arterial access for cardiac catheterization also demonstrated ultrasound significantly decreased the frequency of “difficult access” requiring greater than five attempts or 5 minutes. Given the high incidence of difficult access in critically ill patients, due to peripheral edema, peripheral vascular disease, and weak pulses, ultrasound guidance for arterial cannulation is likely even more valuable in the ICU compared to other settings.
Deep venous thrombosis (DVT) is a common complication in postsurgical and ICU patients that can have serious sequelae including pulmonary embolism. Diagnosis of DVT is traditionally made by a sonographer-performed vascular examination with formal interpretation by a specialist. In contrast, examination with POCUS by a critical care physician can reduce time to diagnosis and be performed when sonographers are unavailable. By using 2D ultrasound to examine the popliteal artery and femoral veins for compressibility along their length, proximal DVTs can be readily diagnosed at the bedside. In one study, a sensitivity and specificity of 86% and 96%, respectively, was achieved even with inexperienced practitioners. Ultrasound screening for DVT by an intensivist is a Grade 1B recommendation in SCCM guidelines.
Pulmonary Ultrasound
Ultrasound examination of the lung can be used to identify and manage many pathologies in the ICU. Normal lung is well aerated and does not transmit ultrasound. When imaging healthy lung, only the pleural line is visualized and anything beyond the pleural line is noise or artifact. However, changes or loss in these characteristic ultrasound artifacts can be used to identify pathology.
Three artifacts are expected in normal lung ultrasound: A-lines, lung sliding, and B-lines. A-lines are regularly spaced repeats of the pleural line that appear as horizontal lines deep to the true pleural line. These are artifacts created by reverberations between pleural line and soft tissue. “Lung sliding” artifact is a shimmering of the pleural line that has been described as “ants walking on a twig” that also causes shifting graininess beyond the pleural line. This is created by the sliding of the parietal and visceral pleura of the lung against each other. The M-mode corollary of lung sliding is the “sandy beach” sign, where the soft tissue above pleura appears as stable horizontal lines, “sky,” and below the pleural line a grainy pattern of “a sandy beach” due to lung sliding artifact. See Fig. 83.1 for an example of M-mode appearance of normal lung. “B-lines” are a third kind of artifact occasionally seen in normal lung. They are vertical streaks that radiate down from the pleural line to the far field image. Sometimes called “comet tails” or “pleural rockets,” they are caused by fine reverberation within interlobular septae. One or two B-lines in a rib space can be normal, especially in the dependent lung fields.
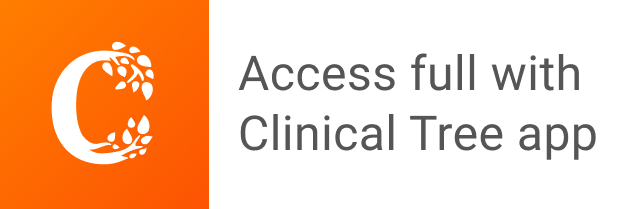