Pearls
- •
The neonatal myocardium is less compliant than that of the older child, less tolerant of increases in afterload, and less responsive to increases in preload. A predictable decrease in cardiac index typically occurs 6 to 12 hours after separation from cardiopulmonary bypass, but milrinone administration during the early postoperative period may attenuate this phenomenon.
- •
Patients with postoperative low cardiac output (CO) require careful evaluation for unanticipated residual lesions.
- •
Patients with restrictive physiology from hypertrophy and diastolic dysfunction of the right ventricle may require high right-sided filling pressures to achieve adequate cardiac output, making them prone to hepatic congestion, anasarca, pleural effusions, and ascites.
- •
Inhaled nitric oxide plays an important role in the management of postoperative pulmonary hypertension in the cardiac intensive care unit.
- •
Hypoxemia after bidirectional cavopulmonary anastomosis generally is a sign of decreased pulmonary blood flow related to reduced cardiac output.
- •
Liberation from positive-pressure mechanical ventilation should be accomplished as soon as feasible, particularly in patients after a cavopulmonary anastomosis (bidirectional Glenn) or Fontan operation because spontaneous breathing improves pulmonary blood flow, arterial oxygen saturation, and ventricular preload.
- •
Ventricular ectopy and elevated atrial pressures after the arterial switch operation should raise suspicion of myocardial ischemia from insufficient coronary blood flow.
- •
Postoperative care of the patient with hypoplastic left heart syndrome after stage I palliation (Norwood procedure) may require delicate balancing of the pulmonary and systemic blood flows. A high arterial oxygen saturation denotes excessive pulmonary blood flow and in patients with impaired ventricular output is generally accompanied by inadequate systemic blood flow, acidosis, and end-organ dysfunction.
Congenital anomalies account for the largest diagnostic category among causes of infant mortality in the United States. Structural heart disease leads the list of congenital malformations. Of the more than 4 million children born each year in the United States, nearly 40,000 have some form of congenital heart disease (CHD). Approximately half of these children appear for therapeutic intervention within the first year of life; the majority require critical care expertise in pediatric intensive care units (PICUs). We recognize that many centers have developed separate specialized pediatric cardiac intensive care units to care for these patients, while some continue to cohort cardiac patients within a general PICU. In this chapter, the PICU designation is used interchangeably to denote the unit caring for critically ill patients necessitating care following surgery or procedures to treat congenital cardiac conditions. These patients now represent a major diagnostic category for admissions in large PICUs across the country, accounting for 30% to 40% or more of PICU admissions in many centers. In addition to the traditional pediatric-age patients, many PICUs now also care for young adult survivors of congenital heart disease, since these patients now outnumber children with congenital heart disease in the general population.
Neonatal considerations
Care of the critically ill neonate requires an appreciation of the special structural and functional features of immature organs, the interactions of the transitional neonatal circulation, and the secondary effects of the congenital heart lesion on other organ systems. The neonate responds more quickly and profoundly to physiologically stressful circumstances, such as rapid changes in pH, lactic acid, blood glucose, and temperature. Neonates have diminished fat and carbohydrate reserves compared with older children; however, they have a higher metabolic rate. Immaturity of the liver and kidney may be associated with reduced protein synthesis and glomerular filtration such that drug metabolism is altered and hepatic synthetic function is reduced. These issues may be compounded by the normal increased total body water of the neonate compared with the older patient, along with the propensity for capillary leakage. This is especially prominent in the immature lung of the neonate, in which the pulmonary vascular bed is nearly fully recruited at rest, and the lymphatic recruitment required to handle elevated mean capillary pressures associated with increases in pulmonary blood flow may be suboptimal. The neonatal myocardium is less compliant than that of the older child, less tolerant of increases in afterload, and less responsive to increases in preload. Younger age also predisposes the myocardium to the adverse effects of cardiopulmonary bypass (CPB) and hypothermic ischemia implicit in support techniques used during cardiac surgery. These factors do not preclude intervention in the neonate but rather simply dictate that extraordinary vigilance be applied to the care of these children and that intensive care management plans account for the immature physiology.
The observed benefits of neonatal reparative operations in patients with two ventricles are numerous ( Box 36.1 ). Elimination of cyanosis and congestive heart failure (CHF) early in life optimizes conditions for normal growth and development. Palliative procedures such as pulmonary artery banding and creation of systemic-to-pulmonary artery shunts do not fully address cyanosis or CHF and may introduce their own set of physiologic and anatomic complications. Examples of improved outcomes with a single reparative operation rather than staged palliation as a newborn are well known and evoke little controversy. Approaches that have been abandoned include banding the pulmonary arteries in truncus arteriosus, staging repair of type B interrupted aortic arch (IAA), and staging repair of transposition of the great arteries with IAA. In other conditions (e.g., severely cyanotic newborn with tetralogy of Fallot [TOF]), the risks and benefits of neonatal repair versus a palliative shunt are debatable.
Early elimination of cyanosis
Early elimination of congestive heart failure
Optimal circulation for growth and development
Reduced anatomic distortion from palliative procedures
Reduced hospital admissions while awaiting repair
Reduced parental anxiety while awaiting repair
Whereas the neonate may be more labile than the older child, there is ample evidence that this age group is more resilient in its response to various forms of stress, including metabolic or ischemic injury. Tolerance of hypoxemia in the neonate is characteristic of many species, and the plasticity of the neurologic system in the neonate is well known. It is the rule rather than the exception that neonates presenting with shock secondary to obstructive left heart lesions can be effectively resuscitated without persistent end-organ impairment. The pliability and mobility of vascular structures in the neonate improve the technical aspects of surgery. Reparative operations in neonates take advantage of normal postnatal changes, allowing more normal growth and development in crucial areas such as myocardial muscle, pulmonary parenchyma, and coronary and pulmonary angiogenesis.
Postoperative pulmonary hypertensive events are more common in the infant who has been exposed to weeks or months of high pulmonary pressure and flow. This is especially true for such lesions as truncus arteriosus, complete atrioventricular (AV) canal defects, and transposition of the great arteries (TGA) with ventricular septal defects (VSDs). Finally, cognitive and psychomotor abnormalities associated with months of hypoxemia or abnormal hemodynamics may be diminished or eliminated by early repair. However, if early reparative surgery results in more exposures to CPB (e.g., repeated conduit changes) and associated adverse effects on cognitive or motor function, then the risk-to-benefit assessment must be modified accordingly.
Preoperative care
Optimal preoperative care involves (1) initial stabilization, airway management, and establishment of adequate vascular access; (2) complete and thorough noninvasive delineation of the anatomic defect(s); (3) initiation/termination of prostaglandin therapy, as appropriate; (4) evaluation and treatment of secondary organ dysfunction, particularly the brain, kidneys, and liver; and (5) cardiac catheterization if necessary, typically for (a) physiologic assessment (e.g., vascular response to oxygen or another pulmonary vasodilator), (b) interventions such as balloon atrial septostomy or valvotomy, or (c) anatomic definition not possible by echocardiography (e.g., coronary artery distribution in pulmonary atresia with intact ventricular septum or delineation of aorticopulmonary collaterals in TOF with pulmonary atresia). Magnetic resonance imaging (MRI) and magnetic resonance angiography have emerged as important adjuvant diagnostic modalities in the evaluation of the cardiovascular system, including qualitative assessments of valve and ventricular function, and quantification of flow, ventricular volume, mass, and ejection fraction. ,
Congenital heart defects can be complex and difficult to categorize or conceptualize. Rather than trying to determine the management for each individual anatomic defect, a physiologic approach can be taken. The following questions should be asked:
- 1.
How does the systemic venous return reach the systemic arterial circulation to maintain cardiac output?
- 2.
What, if any, intracardiac mixing, shunting, or outflow obstruction exists?
- 3.
Are the pulmonary and systemic circulations in series or parallel?
- 4.
Are the defects amenable to a two-ventricle or single-ventricle repair?
- 5.
Is pulmonary blood flow increased or decreased?
- 6.
Is there a volume load or pressure load on the ventricles?
Appropriate organization of preoperative data, patient preparation, and decisions about monitoring, anesthetic agents, and postoperative care are best accomplished by focusing on a few major pathophysiologic problems, beginning with whether the patient is cyanotic, is in CHF, or both. Most pathophysiologic mechanisms that are pertinent to optimal patient preparation and to the perioperative plan focus on one of the following major problems: severe hypoxemia, excessive pulmonary blood flow, CHF, obstruction of blood flow from the left heart, and poor ventricular function. Although some patients with congenital heart disease present with only one of these problems, many have multiple interrelated issues.
Severe hypoxemia
In the first few days of life, many of the cyanotic forms of CHD present with severe hypoxemia (Pa o 2 <50 mm Hg) in the absence of respiratory distress. Infusion of prostaglandin E 1 (PGE 1 ) in patients with decreased pulmonary blood flow maintains or reestablishes pulmonary flow through the ductus arteriosus. This may also improve mixing of venous and arterial blood at the atrial level in patients with transposition of the great arteries. Consequently, neonates rarely require surgery while severely hypoxemic.
PGE 1 dilates the ductus arteriosus of the neonate with life-threatening ductus-dependent cardiac lesions and improves the patient’s condition before surgery. PGE 1 can reopen a functionally closed ductus arteriosus even several days after birth, or it can be used to maintain patency of the ductus arteriosus for several months postnatally. , The common side effects of PGE 1 infusion—apnea, hypotension, fever, central nervous system (CNS) excitation—are easily managed in the neonate when normal therapeutic doses of the drug (0.01–0.05 μg/kg per minute) are used. However, PGE 1 is a potent vasodilator; thus, intravascular volume may require augmentation at higher doses. Patients with intermittent apnea resulting from administration of PGE 1 may require mechanical ventilation preoperatively, although apnea can resolve with the concomitant administration of aminophylline or caffeine. PGE 1 usually improves the arterial oxygenation of hypoxemic neonates who have poor pulmonary perfusion as a result of obstructed pulmonary flow (critical pulmonic stenosis or pulmonary atresia) by providing pulmonary blood flow from the aorta via the ductus arteriosus. The improved oxygenation reverses the lactic acidosis that often develops during episodes of severe hypoxia, and clinical improvement is seen in a matter of minutes to hours.
Excessive pulmonary blood flow
Excessive pulmonary blood flow is frequently the primary problem of patients with CHD. The intensivist must carefully evaluate the hemodynamic and respiratory impact of left-to-right shunts and the extent to which it contributes to the perioperative course in the ICU. Children with left-to-right shunts may have chronic low-grade pulmonary infection and congestion that cannot be eliminated despite optimal preoperative preparation. If so, surgery should not be postponed further. Respiratory syncytial viral infections are particularly prevalent in this population, but advances in intensive care have markedly improved outcomes with this and other viral pneumonias.
Aside from the respiratory impairment caused by increased pulmonary blood flow, the left heart must dilate to accept pulmonary venous return that might be several times normal. If the body requires more systemic blood flow, the neonatal heart responds inefficiently, as most of the increment in cardiac output is recirculated to the lungs. Eventually, symptoms of CHF appear. Medical management with inotropes, systemic vasodilators, and diuretics may improve the patient’s condition, but the diuretics may induce profound hypochloremic alkalosis and potassium depletion that often persist after surgery.
Obstruction of left heart outflow
Patients who require surgery to relieve obstruction to outflow from the left heart are among the most critically ill children in the ICU. These lesions include interruption of the aortic arch, critical coarctation of the aorta, aortic stenosis (AS), and mitral stenosis or atresia as part of the hypoplastic left heart syndrome (HLHS) spectrum. These neonates present with inadequate systemic perfusion and profound metabolic acidosis. The initial pH may be below 7 despite a low partial pressure of arterial carbon dioxide (Pa co 2 ). Systemic blood flow is largely or completely dependent on blood flow into the aorta from the ductus arteriosus; thus, its closure causes a dramatic worsening of the patient’s condition. The patient suddenly becomes critically ill, and survival requires PGE 1 infusion to allow blood flow into the aorta from the pulmonary artery. , PGE 1 infusion improves perfusion and metabolism in neonates with acidosis, metabolic derangements, and renal failure because of inadequate systemic perfusion so that surgery generally can be deferred until stability is achieved. Ventilatory and inotropic support and correction of metabolic acidosis—along with calcium, glucose, and electrolyte abnormalities—should occur preoperatively. Adequacy of stabilization, rather than severity of illness at presentation, appears to influence postoperative outcome the most.
Ventricular dysfunction
Ideally, the intensivist should participate in the preoperative care of all patients who are expected to recover in the ICU after surgery. Understanding the extent of ventricular dysfunction preoperatively provides considerable insight into intraoperative and postoperative events. Although patients with large shunts may have only mild-to-moderate hypoxemia as a result of excessive pulmonary blood flow, the price paid for near-normal arterial oxygen saturation is chronic ventricular dilation and dysfunction and pulmonary vascular obstructive disease. Older patients with CHD and poor ventricular function as a result of chronic ventricular volume overload (aortic or mitral valve regurgitation or longstanding systemic-to-pulmonary arterial shunts) present a different challenge, mitigated to some extent by afterload reduction. However, great care must be exercised in hearts with chronic volume overload as there is a propensity for ventricular fibrillation during sedation, anesthesia, or intubation of the airway. For patients with significantly increased pulmonary-to-systemic flow ratio (Qp/Qs), systemic blood flow should be optimized without further augmenting pulmonary flow during induction of anesthesia in the ICU or in the operating room.
Postoperative care
Assessment
When the clinical course of patients after cardiac surgery deviates from the usual expectation of uncomplicated recovery, our first responsibility is to verify the accuracy of the preoperative diagnosis and the adequacy of surgical repair. For example, a young infant who is acidotic, hypotensive, and cyanotic after surgical repair of TOF may tempt us to ascribe these findings to the vagaries of ischemia/reperfusion injury caused by CPB or transient, postoperative stiffness of the right ventricle. However, the real culprit may be an additional VSD undetected preoperatively and therefore not closed, a significant surgical patch leak, or residual right ventricle (RV) outflow obstruction. Correct postoperative assessment is imperative, and treatment follows accordingly. Evaluation of the postoperative patient relies on examination, monitoring, interpretation of vital signs, and imaging. Only when the accuracy of the diagnosis and adequacy of the repair are established can a CPB-related low–cardiac output state be presumed and treatment optimized. Treating low–cardiac output states and preventing cardiovascular collapse often are the central features of pediatric cardiac intensive care and are the focus of this chapter ( Box 36.2 ). The details of the specific considerations for selected lesions are presented in their respective sections.
- 1.
Know in detail the cardiac anatomy and its physiologic consequences.
- 2.
Understand the specialized considerations of the newborn and implications of reparative rather than palliative surgery.
- 3.
Diversify personnel to include experts in neonatal and adult congenital heart disease.
- 4.
Monitor, measure, and image the heart to rule out residual disease as a cause of postoperative hemodynamic instability or low cardiac output.
- 5.
Maintain aortic perfusion and improve the contractile state.
- 6.
Optimize preload (including atrial shunting).
- 7.
Reduce afterload.
- 8.
Control heart rate, rhythm, and synchrony.
- 9.
Optimize heart-lung interactions.
- 10.
Provide mechanical support when needed.
The initial assessment following cardiac surgery begins with a review of the operative findings. This includes details of the operative repair and CPB, particularly total CPB time, myocardial ischemia (aortic cross-clamp), and circulatory arrest or antegrade perfusion times; concerns about myocardial protection; recovery of myocardial contractility; typical postoperative systemic arterial and central venous pressures; findings from intraoperative transesophageal or epicardial echocardiography, if performed; vasoactive medication requirements; and hemostatic management. This information guides subsequent examination, which should focus on the quality of the repair or palliation plus a clinical assessment of cardiac output ( Box 38.3 ). In addition to a complete cardiovascular examination immediately upon arrival to the PICU, a routine set of laboratory tests should be obtained, including a chest radiograph, 12- or 15-lead electrocardiography (ECG), blood gas analysis, serum electrolytes and glucose, ionized calcium and lactate measurements, complete blood count, and coagulation profile.
Signs
Cool extremities/poor perfusion
Oliguria and other end-organ failure
Tachycardia
Hypotension
Acidosis
Cardiomegaly
Pleural effusions
Monitor and assess
Heart rate, blood pressure, intracardiac pressure
Extremity temperature, central temperature
Urine output
Mixed venous oxygen saturation
Arterial blood gas pH and lactate
Laboratory measures of end-organ function
Echocardiography
This information, together with an understanding of the preoperative and postoperative loading conditions of the heart, are essential for clinical management in the immediate postoperative period. Optimizing preload involves more than just giving volume to a hypotensive patient. There are numerous considerations to fluid balance involving types of isotonic fluid, ultrafiltration in the operating room, optimal hematocrit, and the use of diuretics or vasopressors. Fluid itself can be detrimental if excess extravascular water results in interstitial edema and end-organ dysfunction of vital organs such as the heart, lungs, and brain. Occasionally, permitting a right-to-left shunt at the atrial level can optimize preload to the left ventricle in some conditions (discussed later). Maintaining aortic perfusion after CPB and improving the contractile state of the heart with higher doses of catecholamines are reasonable goals, but they may have particularly deleterious consequences in the newborn myocardium after hypothermic CPB. The benefits of afterload reduction are well known but, if excessive, may result in hypotension, coronary insufficiency, and cardiovascular collapse. Pacing the heart can stabilize the rhythm and hemodynamics, but it also may contribute to dyssynchronous, inefficient cardiac contraction or may induce other arrhythmias. Although lifesaving in many instances, mechanical support of the failing myocardium in the form of extracorporeal life support (ECLS) or ventricular assist devices has its own set of limitations and morbidities. Almost every treatment approach has its own set of adverse effects. Supporting cardiac output in the postoperative patient is a balance between the promise and poison of therapy.
Monitoring
The goal of postoperative monitoring following cardiac surgery primarily focuses on assessing the adequacy of circulatory status and oxygen delivery. The level of vigilance in the immediate postoperative period can be optimized by the combination of laboratory values and physical examination findings with data obtained via noninvasive devices and invasive monitoring to assess intracardiac pressures and oxygen saturations.
Near-infrared spectroscopy (NIRS) provides a noninvasive, continuous estimate of regional oxygen supply and demand that serves as a surrogate for hemodynamics and cerebral and somatic oxygenation. It is based on the differential absorption of varying wavelengths of light by hemoglobin as it associates with oxygen to measure oxygen content in a localized tissue bed. While data demonstrating a definitive impact on overall outcome are lacking, there are reports that correlate decreased cerebral and/or somatic NIRS with increased mortality, prolonged length of stay (LOS), and worsening neurologic outcomes. ,
Invasive monitoring of central venous pressure is routine for most patients following cardiac surgery. Intracardiac or transthoracic left atrial (LA) catheters are often used to monitor patients after complex reparative procedures. Pulmonary arterial (PA) catheters now are seldom used but may be particularly useful if one anticipates postoperative pulmonary hypertension, allowing rapid detection of pressure changes and assessment of the response to interventions.
LA catheters are especially helpful in the management of patients with ventricular dysfunction, coronary artery perfusion abnormalities, or mitral valve disease. The mean LA pressure typically is 1 to 2 mm Hg greater than mean right atrial (RA) pressure, which generally varies between 1- and 6-mm Hg in nonpostoperative pediatric patients undergoing cardiac catheterization. In postoperative patients, mean LA and RA pressures are often greater than 6 to 8 mm Hg. However, they generally should be less than 15 mm Hg. The compliance of the right atrium is greater than that of the left atrium except in the newborn; thus, pressure elevations in the right atrium of older patients with two ventricles typically are less pronounced. Possible causes of abnormally elevated LA pressure are listed in Box 38.4 .
Decreased ventricular systolic or diastolic function
Left atrioventricular valve disease
Large left-to-right intracardiac shunt
Chamber hypoplasia
Intravascular or ventricular volume overload
Cardiac tamponade
Arrhythmia
In addition to pressure data, intracardiac catheters in the right atrium (or a percutaneously placed central venous catheter), left atrium, and pulmonary artery can be used to monitor the oxygen saturation of systemic or pulmonary venous blood and indicate the presence or absence of atrioventricular synchrony. Following reparative surgery, patients with no intracardiac shunts and adequate cardiac output may have a mild reduction in RA oxygen saturation to approximately 60%. Lower RA oxygen saturation does not necessarily indicate low cardiac output. If a patient has arterial desaturation (complete mixing lessons, lung diseases, and so on), the arteriovenous oxygen saturation difference is normally <30%. Hence, even a low RA saturation may be in keeping with appropriate oxygen delivery and extraction. Elevated RA oxygen saturation often is the result of left-to-right shunting at the atrial level (e.g., from the left atrium, anomalous pulmonary vein, or left ventricular [LV]-to-RA shunt). Blood in the LA normally is fully saturated with oxygen (i.e., approximately 100%). The two chief causes of reduced LA oxygen saturation are an atrial-level right-to-left shunt and pulmonary venous desaturation from abnormal gas exchange.
In the absence of left-to-right shunts, PA oxygen saturation is the best representation of the “true” mixed venous oxygen saturation because all sources of systemic venous blood should be thoroughly mixed as they are ejected from the RV. When elevated, this saturation is useful in identifying residual significant left-to-right shunts following repair of a VSD. The absolute value of the PA oxygen saturation is a predictor of significant postoperative residual shunt. In patients following TOF or VSD repair, PA oxygen saturation greater than 80% within 48 hours of surgery with a fractional inspired oxygen concentration (Fi o 2 ) less than 0.5 is a sensitive indicator of significant left-to-right shunt (Qp/Qs >1.5) 1 year after surgery.
Low cardiac output syndrome
Although low cardiac output after CPB is often attributable to residual or undiagnosed structural lesions, progressive low–cardiac output states do occur. A number of factors have been implicated in the development of myocardial dysfunction following CPB, including (1) the inflammatory response associated with CPB, (2) myocardial ischemia from aortic cross-clamping, (3) hypothermia, (4) reperfusion injury, (5) inadequate myocardial protection, and (6) ventriculotomy (when performed). The typical decrease in cardiac index has been well characterized in newborns following an arterial switch operation (ASO). In a group of 122 newborns, the median maximal decrease in cardiac index that typically occurred 6 to 12 hours after separation from CPB was 32%, and 1 in 4 of these newborns reached a nadir of the cardiac index lower than 2 L/min per square meter. Anticipation of this low cardiac output syndrome (LCOS) and appropriate intervention can do much to avert morbidity or the need for mechanical support. Mixed venous oxygen saturation, whole-blood pH, and lactate are laboratory measures commonly used to evaluate the adequacy of tissue perfusion and, hence, cardiac output.
Volume adjustments
After CPB, the factors that influence cardiac output—such as preload, afterload, myocardial contractility, heart rate, and rhythm—must be continuously assessed and manipulated as needed. Volume expansion (increased preload) is commonly necessary, followed by appropriate use of vasoactive agents. Atrial pressure and the ventricular response to changes in atrial pressure must be evaluated. Ventricular response is judged by observing systemic arterial pressure and waveform, heart rate, skin color, peripheral extremity temperature, peripheral pulse magnitude, urine output, core body temperature, and acid-base balance.
Preserving and creating right-to-left shunts
Selected children with low cardiac output may benefit from strategies that allow right-to-left shunting at the atrial level in the face of expected postoperative RV dysfunction. A typical example is early repair of TOF, when the hypertrophied and poorly compliant right ventricle may be further compromised by increased volume load from pulmonary regurgitation secondary to a transannular patch on the RV outflow tract. These children will benefit from leaving the foramen ovale patent to permit right-to-left shunting of blood, thus preserving cardiac output and oxygen delivery despite the attendant transient cyanosis. When the foramen ovale is not patent or is surgically closed, RV dysfunction can lead to reduced LV filling, low cardiac output, and, ultimately, LV dysfunction. In infants and neonates with repaired truncus arteriosus, the same concerns apply and may even be exaggerated if RV afterload is elevated because of pulmonary hypertension.
Other strategies
Additional strategies to support low cardiac output associated with cardiac surgery in children include the use of atrioventricular pacing for patients with complete heart block or prolonged interventricular conduction delays and asynchronous contraction. Appreciation of the hemodynamic effects of positive and negative pressure ventilation may be used to assist cardiac output. Avoidance of elevated body temperatures and even inducing hypothermia along with appropriate sedation and even paralysis may provide end-organ protection during periods of low cardiac output and aid in the management of postoperative arrhythmias, such as junctional ectopic tachycardia.
Mechanical cardiac support
Perioperative mechanical circulatory support (MCS) can be lifesaving for critically ill children and young adults with CHDs. , The most common form of pediatric MCS is extracorporeal membrane oxygenation (ECMO). ECMO can be used as rescue during extracorporeal cardiopulmonary resuscitation (ECPR), in failure to wean from CPB, or in patients who develop low–cardiac output states postoperatively despite high levels of pharmacologic support. , it is presently less commonly used as a bridge to heart transplantation—it is more often used as a prelude to long-term mechanical assist devices or simply to allow time for decision-making. , An analysis of 96,596 operations from 80 centers reporting to the Society of Thoracic Surgeons Congenital Heart Surgery Database showed that MCS was used in 2.4% of cases. Children who underwent Norwood procedures (17%) or complex biventricular repairs (14%) were more likely to receive MCS. Substantial variation exists in MCS rates across both high- and low-volume centers. Overall, 53% of those children who received MCS did not survive to hospital discharge, with mortality greater than 70% for certain operative lesions (truncus arteriosus repair, Ross-Konno operation). Despite this high mortality, it is important to recognize that survival would have been virtually zero for those children without MCS.
In a recent report from the Pediatric Cardiac Critical Care Consortium Registry, of the 14,526 eligible medical as well as surgical cardiac ICU hospitalizations, 449 (3.1%) had at least one ECMO run. Of these, 329 (3.5%) were surgical and 120 (2.4%) were medical hospitalizations. Of the surgical group, 33 (10%) included preoperative ECMO only and 296 (90%) included postoperative ECMO. Overall, in-hospital mortality was 48.9% in the surgical group and 63.3% in the medical group; mortality rates for hospitalizations including ECPR were 82.7% (surgical) and 50% (medical).
Due to improved technology, reliability, and mechanical durability of devices, higher rates of patient survival, reduced adverse events, and limited availability of organs for transplantation, intracorporeal MCS has become an accepted long-term inpatient and outpatient therapy for those with advanced heart failure awaiting heart transplantation. Ventricular assist devices (VADs) can support function of the left ventricle (left ventricular assist device [LVAD]), right ventricle (right ventricular assist device [RVAD]), or both (biventricular assist device [BiVAD]). A total artificial heart (TAH) replaces the heart itself. VADs have two different mechanisms of blood flow: pulsatile or continuous. Continuous flow devices contain an impeller that rotates at high speed to propel blood. These include axial flow impellers (e.g., HeartMate II [Thoratec Corp.]), or centrifugal pumps (e.g., HeartWare HVAD [HeartWare Corp.]). Paracorporeal pulsatile devices (e.g., Thoratec PVAD-BiVAD [Thoratec Corp.], Berlin Heart EXCOR BiVAD [Berlin Heart Corp.]) are also used in selected cases. Guidelines exist with regard to CPR in children with MCS. A more detailed discussion of MCS can be found in Chapter 28 .
Right ventriculotomy and restrictive physiology
Right ventricular restrictive physiology has been demonstrated by echocardiography as persistent anterograde diastolic blood flow into the pulmonary circulation following reconstruction of the RV outflow in infants and children. This occurs in the setting of elevated RV end-diastolic pressure and RV hypertrophy when the right ventricle demonstrates diastolic dysfunction with an inability to properly relax and fill during diastole. The poorly compliant RV usually is not dilated in this circumstance, and pulmonary valve regurgitation is limited because of the elevated RV diastolic pressure. ,
The term restrictive RV physiology is also commonly used in the immediate postoperative period in patients who have a stiff, poorly compliant, and sometimes hypertrophied RV. The elevated ventricular end-diastolic pressure restricts filling during diastole, causing an increase in RA filling pressure and, ultimately, systemic venous hypertension. Because of the phenomenon of ventricular interdependence, changes in RV diastolic function and septal position in turn affect LV compliance and function. Factors contributing to diastolic dysfunction include lung and myocardial edema following CPB, inadequate myocardial protection of the hypertrophied ventricle during aortic cross-clamp, coronary artery injury, residual outflow tract obstruction, volume load on the ventricle from a residual VSD or pulmonary regurgitation, and dysrhythmias. In many centers, a residual atrial communication is left to mitigate the perioperative sequelae associated with restrictive RV physiology, namely, a low–cardiac output state. In such a scenario, patients may be desaturated following surgery (typically in the 75% to 85% range) because of this right-to-left shunting, but they maintain systemic cardiac output while avoiding significant systemic venous hypertension. As RV compliance and function improve (usually within 2 to 3 postoperative days), the amount of shunt decreases and both anterograde pulmonary blood flow and Sa o 2 increase.
If significant restrictive RV physiology develops in the absence of an unrestrictive atrial communication, a low–cardiac output state with increased right-sided filling pressure (usually >12 mm Hg) ensues. Such patients often have cool extremities, oliguria, and metabolic acidosis. As a result of the elevated RA pressure, hepatic congestion, ascites, increased chest tube output, and pleural effusions may be evident. These patients may be tachycardic and hypotensive, with a narrow pulse pressure. Preload must be maintained despite an already elevated RA pressure. Significant inotropic support often is required (typically, epinephrine 0.05–0.1 µg/kg per minute). A phosphodiesterase inhibitor, such as milrinone, can be beneficial because of its lusitropic properties; however, one must be cautious in the use of these agents with renal impairment. Sedation and paralysis often are necessary for the first 24 to 48 hours to minimize energy expenditure and associated myocardial work. Factors that further impair ventricular diastolic filling—such as loss of AV synchrony, accumulation of pleural fluid or ascites, and high tidal volume ventilation with air trapping—should be mitigated early in the postoperative course.
Mechanical ventilation, either hypoinflation or hyperinflation of the lung, hypothermia, and acidosis can contribute to increased afterload on the right ventricle and pulmonary regurgitation. Synchronized intermittent positive-pressure ventilation with the lowest possible mean airway pressure should be the aim, as discussed previously.
Diastolic dysfunction
Occasionally, there is an alteration of ventricular relaxation, an active energy-dependent process, which reduces ventricular compliance. This is particularly problematic in patients with a hypertrophied ventricle undergoing surgical repair, such as TOF or Fontan surgery, and following CPB in some neonates when myocardial edema may significantly restrict diastolic function. , The poorly compliant ventricle with impaired diastolic relaxation has a reduced end-diastolic volume and stroke volume. β-Adrenergic antagonists and calcium channel blockers add little to the treatment of this condition. In fact, hypotension or myocardial depression produced by these agents often outweighs any gain from slowing the heart rate. Calcium channel blockers are relatively contraindicated in neonates and small infants because of their dependence on transsarcolemmal flux of calcium to both initiate and sustain contraction.
A gradual increase in intravascular volume to augment ventricular capacity, in addition to the use of low doses of inotropic agents, is of modest benefit in patients with diastolic dysfunction. Tachycardia must be avoided and AV synchrony maintained to optimize diastolic filling time and decrease myocardial oxygen demands. If low cardiac output persists despite treatment, vasodilators can be carefully attempted to alter systolic wall tension (afterload) and thus decrease the impediment to ventricular ejection. Because the capacity of the vascular bed increases after vasodilation, simultaneous volume replacement is often necessary. A noncatecholamine inodilator with vasodilating and lusitropic (improved diastolic state) properties, such as milrinone, is useful under these circumstances in contrast with other inotropic agents.
Pharmacologic support
General principles of pharmacologic support in the neonatal and pediatric patient center on the recognition of the developmental limitations of the neonatal myocardium and the well-described reductions in cardiac output 6 to 12 hours after separation from CPB. Despite ongoing development and maturity of adrenergic receptors and L-type calcium channels, catecholamine-based inotropic agents and vasodilators are efficacious in this population. Other nonvasoactive agents serve as adjuncts to optimizing postoperative hemodynamics and fluid balance. The combination of a low-dose inotrope and an afterload reducing agent—or, more commonly, a phosphodiesterase inhibitor—has been shown to decrease the occurrence of postoperative LCOS following CPB.
It should be understood that the need for vasoactive and inotropic support varies greatly among patients recovering from cardiac surgery and even over time for an individual patient progressing through the postoperative care continuum. The intensity of pharmacologic support employed must be constantly evaluated. One must not embrace a false sense of security when caring for a patient with adequate cardiac output when this requires disproportionate pharmacologic support. The current approach is to employ the lowest level of inotropic and vasoactive support necessary for the achievement of hemodynamic goals. Due to advances in preoperative stabilization, anesthetic strategies, surgical technique, myocardial protection, and CPB, it is not uncommon for a patient to return to the PICU requiring only low doses of milrinone or epinephrine or no pharmacologic support at all. A detailed discussion of cardiovascular pharmacology is beyond the scope of this chapter but can be found in Chapter 31 .
Managing acute pulmonary hypertension in the intensive care unit
Children with many forms of CHD are prone to perioperative elevations in pulmonary vascular resistance (PVR). This situation complicates the postoperative course when transient myocardial dysfunction is further challenged by increased RV afterload.
Although postoperative patients with pulmonary hypertension often are presumed to have active and reversible pulmonary vasoconstriction as the source of their pathophysiology, the intensivist is obligated to explore anatomic causes of mechanical obstruction that impose a barrier to pulmonary blood flow. Elevated LA pressure, pulmonary venous obstruction, branch pulmonary artery stenosis, or surgically induced loss of the vascular tree all raise RV pressure and impose an unnecessary burden on the right heart. Similarly, a residual or undiagnosed left-to-right shunt raises pulmonary artery pressure postoperatively and must be addressed surgically. In these cases, the use of pulmonary vasodilator strategies augments only residual or undiagnosed shunts and increases the volume load on the heart.
Several factors peculiar to CPB may raise PVR: pulmonary vascular endothelial dysfunction, microemboli, pulmonary leukostasis, excess thromboxane production, atelectasis, hypoxic pulmonary vasoconstriction, and adrenergic events all have been suggested to play a role in postoperative pulmonary hypertension. Postoperative pulmonary vascular reactivity has been related not only to the presence of preoperative pulmonary hypertension and left-to-right shunts but also to the duration of total CPB. The threat of postoperative pulmonary hypertensive crises can be partially addressed by providing surgery at earlier ages, pharmacologic interventions, and other postoperative management strategies ( Table 36.1 ).
Encourage | Avoid |
---|---|
Anatomic investigation | Residual anatomic disease |
Opportunities for right-to-left shunt as pop-off | Intact atrial septum in right heart failure |
Sedation/anesthesia | Agitation/pain |
Moderate hyperventilation | Respiratory acidosis |
Moderate alkalosis | Metabolic acidosis |
Adequate inspired oxygen | Alveolar hypoxia |
Normal lung volumes | Atelectasis or overdistension |
Optimal hematocrit | Excessive hematocrit |
Inotropic support | Low output and coronary perfusion |
Vasodilators | Vasoconstrictors/increased afterload |
Pulmonary vasodilators
Nitric oxide (NO) is the mainstay of therapy in patients with pulmonary hypertension requiring critical care. NO is a vasodilator formed by the endothelium from l -arginine and molecular oxygen in a reaction catalyzed by NO synthase. NO then diffuses to the adjacent vascular smooth muscle cells, where it induces vasodilation through a cyclic guanosine monophosphate-dependent pathway. , Because NO exists as a gas, it can be delivered by inhalation to the alveoli and, hence, to the adjacent blood vessels. Once it diffuses across the wall of the pulmonary blood vessels, NO enters the vascular lumen. There, it is rapidly inactivated by hemoglobin, resulting in selective pulmonary vasodilation. Inhaled NO (iNO) has advantages over intravenously administered vasodilators that may cause systemic hypotension and increase intrapulmonary shunting. Inhaled NO lowers pulmonary artery pressure in a number of diseases without the unwanted effect of systemic hypotension. This effect is especially dramatic in children with cardiovascular disorders and postoperative patients with pulmonary hypertensive crises. , ,
Therapeutic uses of iNO in children with CHD abound in the ICU. Newborns with total anomalous pulmonary venous connection (TAPVC) frequently have obstruction of the pulmonary venous pathway where it connects anomalously to the systemic venous circulation. When pulmonary venous return is obstructed preoperatively, pulmonary hypertension is severe and requires urgent surgical relief. Use of iNO in this or other settings of suspected pulmonary venous obstruction is contraindicated. On the other hand, increased neonatal pulmonary vasoreactivity, endothelial injury induced by CPB, and remodeling of the pulmonary vascular bed in this disease contribute to postoperative pulmonary hypertension. Postoperatively in the patient with TAPVC after adequate surgical relief of obstruction, iNO dramatically reduces pulmonary hypertension without adverse changes in heart rate, systemic blood pressure, or vascular resistance.
Postoperative patients with TAPVC, congenital mitral stenosis, and other pulmonary venous hypertensive disorders associated with low cardiac output are among the most responsive to iNO. These infants are born with significantly increased amounts of smooth muscle in their pulmonary arterioles and venules. Histologic evidence of muscularized pulmonary veins and pulmonary arteries suggests the presence of vascular tone and capacity for change in resistance at both the arterial and venous sites. The increased responsiveness to iNO seen in younger patients with pulmonary venous hypertension may result from pulmonary vasorelaxation at a combination of precapillary and postcapillary vessels. Resolving the primary venous obstruction is of utmost importance before using iNO in these lesions.
Several groups have reported successful use of iNO in a variety of other congenital heart defects following cardiac surgery. Inhaled NO is especially helpful when administered during a pulmonary hypertensive crisis. Successful iNO use has been described after the Fontan procedure, following late VSD repair, and with a variety of other anatomic lesions for which patients are at risk of developing postoperative pulmonary hypertensive crises. Oxygen saturation in response to iNO generally does not improve in very young infants who are excessively cyanotic after a bidirectional Glenn anastomosis. In these cases, increasing cardiac output and cerebral blood flow will have a much greater impact on arterial oxygenation because elevated pulmonary vascular tone is seldom the limiting factor in the hypoxemic patient after the bidirectional Glenn operation.
Inhaled NO can be used diagnostically in neonates with RV hypertension after cardiac surgery to discern those with reversible vasoconstriction. In patients with Ebstein anomaly, a clinical response to iNO can accurately differentiate between functional and anatomic pulmonary atresia. In addition, the use of iNO in such patients can facilitate anterograde pulmonary blood flow and hemodynamic stabilization. Failure of the postoperative newborn to respond to iNO should be regarded as strong evidence of anatomic and possibly surgically remediable obstruction.
If iNO must be discontinued before the pathologic process has been resolved, hemodynamic instability can be expected. The withdrawal response to iNO can be attenuated by pretreatment with the type V phosphodiesterase inhibitor sildenafil. Sildenafil inhibits the inactivation of cyclic guanosine monophosphate within the vascular smooth muscle cell and has the potential to augment the effects of either endogenous or exogenously administered NO to affect vascular smooth muscle relaxation. Sildenafil can be administered in an oral or intravenous (IV) form and has a somewhat selective pulmonary vasodilating capacity while lowering LA pressure and providing a modest degree of systemic afterload reduction in some postoperative children. Chronic oral administration of sildenafil to adults with primary pulmonary hypertension improves exercise capacity. This phenomenon has also been demonstrated in pediatric patients with a Fontan circulation, perhaps suggesting a broad therapeutic application on older patients after operation for CHD.
Many other vasodilators have been used with variable success in patients with pulmonary hypertensive disorders requiring critical care. IV vasodilators—such as tolazoline, phenoxybenzamine, nitroprusside, and isoproterenol—have little biological basis for selectivity or enhanced activity in the pulmonary vascular bed. However, if myocardial contractility is depressed and the afterload reducing effect on the left ventricle is beneficial to myocardial function and cardiac output, then these drugs may be of some value. In addition to drug-specific side effects, intravenous vasodilators all have the potential to produce profound systemic hypotension, critically lowering coronary perfusion pressure while simultaneously increasing intrapulmonary shunt, thus limiting their usefulness in the management of acute postoperative pulmonary hypertension. In fact, in patients with idiopathic pulmonary hypertension who have adequate LV contractility, the use of a vasopressor may help the RV coronary perfusion pressure, LV preload and systolic interventricular dependence, thus preventing a pulmonary hypertensive crisis.
Management of postoperative bleeding
Infants and children undergoing cardiac surgery are at high risk for hemorrhage and need for transfusion. CPB is a major thrombogenic stimulus that causes a multifactorial coagulopathy due to dilution and consumption of clotting factors and inactivation of platelets. This is further exacerbated by an immature coagulation system and the presence of hypoxia and hypothermia. While upward of 79% of children undergoing cardiac surgery will require transfusion, the need for transfusion is associated with increased morbidity. , Transfusion criteria for packed red blood cells is determined by balancing the inherent risks associated with transfusion and the need to optimize oxygen delivery in the face of hemodynamic instability. However, there are increasing data suggesting that the use of more restrictive transfusion parameters results in less transfusion with no difference in clinical outcome. To minimize the need for excessive blood products, careful attention to ongoing bleeding and coagulopathy is necessary. Baseline complete blood count and coagulation profile should be measured on return from the operating room. Chest tube outputs of 10 mL/kg in the first hour or 5 mL/kg per hour for subsequent hours should prompt aggressive repletion of abnormal clotting factors, initially with platelets and fresh-frozen plasma (FFP), assessment of adequate reversal of anticoagulants, and a discussion with the surgeon. When bleeding is resistant to therapy, transfusion of factor concentrates should be considered. These include fibrinogen concentrate, prothrombin complex concentrates, or even activated factor VII in selected patients. Significant repletion of red blood cells also necessitates the concurrent transfusion of platelets and FFP to avoid further dilution of clotting components. Ongoing chest tube output that does not abate despite normalization of factors suggests the possibility of surgical bleeding that could necessitate reexploration. Further, while control of postoperative bleeding is the goal, the abrupt cessation of chest tube output—particularly when accompanied by increasing CVP, tachycardia, and hypotension—suggests evolving tamponade. Ensuring chest tube patency may be sufficient to reverse the process. If not, reexploration of the mediastinum may be necessary.
Cardiac tamponade
The infant’s crowded mediastinum makes compression of the heart and cardiac tamponade an ever-present possibility after chest closure, despite patent drainage tubes and surgical resection of the anterior pericardium. The warning signs of tamponade frequently are subtle in small children, even minutes before cardiovascular collapse. Any significant deterioration in hemodynamics after chest closure should first be attributed to tamponade if ventilation and cardiac rhythm are adequate. The signs of tamponade include tachycardia, hypotension, narrow pulse pressure, and high filling pressures on both the left and right sides of the heart.
Acute myocardial perforation with tamponade occasionally occurs during interventional cardiac catheterization procedures. Prompt support of the circulation with volume infusions and pressor support, along with immediate catheter drainage of the pericardial space, are essential in the event of this complication. Hemopericardium after ventricular puncture usually is self-limited, as the muscular ventricle seals the perforation after the responsible wire or catheter is removed. However, laceration of the thin-walled atrium may require suture repair under direct vision in the operating room.
Other causes of cardiac tamponade are seen in patients with CHD; treatment frequently requires the assistance of an intensivist for either pericardiocentesis or sedation and monitoring for that definitive procedure. Postoperative tamponade from bleeding immediately after operation, as discussed earlier, is best handled by facilitation of chest tube drainage or reopening the sternotomy. Some children develop pericardial effusions during later phases of their illness because of hydrostatic influences (e.g., patients with modified Fontan operations) or postpericardiotomy syndrome. Fluid in the pericardial space may accumulate under considerable pressure and to the point at which filling of the heart is impaired. If this problem is left unattended, the transmural pressure in the atria diminishes as intraatrial pressures rise, and diastolic collapse of the atria can be observed echocardiographically. Patients become symptomatic with a narrow pulse pressure, pulsus paradoxus, tachycardia, respiratory distress, decreased urine output, hyperkalemia, metabolic acidosis, and hypotension with tremendous endogenous catecholamine response.
Diaphragmatic dysfunction, effusions, and pulmonary issues
Diaphragmatic paresis (reduced motion) or paralysis (paradoxical movement) may precipitate and promote respiratory failure, particularly in the neonate or young infant who largely relies on diaphragmatic function for breathing; older infants and children can recruit accessory and intercostal muscles if diaphragmatic function proves inadequate. Injury to the phrenic nerve may occur during operations that require dissection of the branch pulmonary arteries well out to the hilum (e.g., TOF repair, ASO), arch reconstruction from the midline (e.g., Norwood operation), manipulation of the superior vena cava (SVC; Glenn shunt), takedown of a systemic-to-pulmonary shunt, or after attempted percutaneous central venous access. Phrenic nerve injury occurs more frequently at reoperation, when adhesions and scarring may obscure anatomic landmarks. Extensive thymectomy during neonatal operations to improve exposure also can result in phrenic nerve injury. Topical cooling with ice during deep hypothermia may cause transient phrenic palsy. Increased work of breathing on low ventilator settings, increased Pa co 2 , and a chest radiograph revealing an elevated hemidiaphragm suggest diaphragmatic dysfunction. However, the chest radiograph may be misleading if it is obtained at the end of inspiration during positive-pressure ventilation when lung volume is at its highest. Ultrasonography is most useful for identifying reduced diaphragmatic motion or paradoxical excursion. Diaphragmatic dysfunction may be transient and resolve over time. However, a patient who fails repeated extubation attempts despite optimizing cardiovascular and nutritional status, and in whom diaphragmatic dysfunction persists with lung volume loss in the affected side, necessitates surgical plication of the diaphragm. Although only a temporary effect is gained from plication, the prevention of collapse and volume loss in the affected lung from paradoxical movement of the diaphragm often provides the critical advantage needed for liberation from positive-pressure ventilation.
Pleural effusions and ascites may occur in patients after any type of cardiothoracic surgical procedures, especially following the Fontan operation or repairs involving a right ventriculotomy (e.g., TOF, truncus arteriosus) with transient RV dysfunction. Especially in young patients, pleural effusions and increased interstitial lung water may be a manifestation of right heart failure. This seems logically related to raised systemic venous pressure impeding lymphatic return to the venous circulation. Pleural or peritoneal fluid and intestinal distension compete with intrapulmonary gas for thoracic space. Evacuation of the pleural space, drainage of ascites, and bowel decompression facilitate restoration of lung volume.
Pulmonary edema, pneumonia, and atelectasis are common causes of abnormal postoperative gas exchange and hypoxemia. If a bacterial pathogen is identified in the respiratory secretions, antibiotics should be initiated promptly. If pulmonary edema is responsible for the gas exchange abnormality, therapy is aimed at lowering the LA pressure through diuresis and pharmacologic means to reduce afterload and improve the lusitropic state of the heart. For infants, fluid restriction frequently is incompatible with adequate nutrition; therefore, an aggressive diuretic regimen is preferable to restriction of caloric intake. Adjustment of end-expiratory pressure and mechanical ventilation serve as supportive therapies until the alveoli and pulmonary interstitium are cleared of the fluid that interferes with gas exchange.
Chylothorax
Chylothorax develops in 0.25% to 9.2% of children after cardiac surgery and is associated with negative outcomes, including longer LOS, higher hospitalization costs, and increased risk of in-hospital mortality. The etiologies and pathophysiology of lymphatic dynamic disorders in these children are poorly understood, but new insights are emerging.
The thoracic duct ascends to the right of the vertebral column, crosses over to the left hemithorax at the fifth thoracic vertebral body, and drains into the venous circulation at the region of the left subclavian and left jugular veins. In general, chylothorax can be classified as traumatic or nontraumatic. Direct injury to the thoracic duct or its tributaries causes traumatic chylothorax, whereas processes that elevate the central venous pressure (e.g., RV diastolic dysfunction, Fontan physiology, thrombosis or obstruction of the subclavian or internal jugular veins) may lead to nontraumatic chylothorax from alterations in the Starling forces.
Multiple diagnostic and therapeutic algorithms have been reported in the literature. , Initial investigation includes a chest radiograph or ultrasound to confirm the presence of an effusion, followed by diagnostic and/or therapeutic thoracentesis with pleural fluid analysis and an echocardiogram. Vascular ultrasound imaging or cardiac catheterization may be needed to delineate the etiology further in select cases. Typically, chylothorax should be suspected when a “milky” exudate or unilateral effusion is noted in the postoperative period, classically after enteral feeding is resumed. However, a milky appearance alone is insufficient to diagnose chylothorax. Fluid triglyceride levels and cell count with differential are required to further establish the diagnosis. Fluid triglycerides greater than 110 mg/dL or less than 50 mg/dL essentially confirm or exclude the diagnosis, respectively; uncertain cases with values 50 to 110 mg/dL may require additional testing, such as lipoprotein analysis for the demonstration of chylomicrons. In children who are not on enteral feeds or are malnourished, a lipoprotein analysis is suggested even with triglycerides less than 50 mg/dL. Typical pleural fluid in chylothorax has a white cell count greater than 1000/μL with lymphocytic predominance (>80%), and a low lactate dehydrogenase level. In addition, chyle has a high protein (>20 g/L) and immunoglobulin content. , Atypical fluid characteristics—such as transudates, neutrophil-predominance, or high lactate dehydrogenase measurement—signal another etiology, such as heart, liver, or kidney dysfunction, or infection.
Prolonged chylothorax increases the risk of infection, poor wound healing, malnutrition, fluid and electrolyte imbalances, and delayed separation from respiratory support, all of which may lead to worse outcomes. , Management of postoperative chylothorax can be challenging and includes both conservative and interventional treatments, with considerable institutional practice variation. , In general, treatment begins with the insertion of a chest tube to drain the effusion, confirm the diagnosis, and provide symptomatic relief. Postoperative chylothorax can be divided into low volume (≤20 mL/kg per day) or high volume (>20 mL/kg per day) output. Children with low-volume chylothorax are generally started on a high medium-chain triglyceride (MCT), low long-chain triglyceride diet for 7 days. The high MCT diet is continued for 6 weeks in those patients who respond with a decrease in output to less than 10 mL/kg per day. Those who fail this initial dietary modification or have high-volume chylothorax are generally treated with enteral fasting and parenteral nutrition for 7 to 10 days, with consideration for concomitant initiation of somatostatin or its synthetic analog octreotide, administered intravenously or subcutaneously. , Absence of response to this strategy after 2 weeks should prompt consideration of surgical exploration to identify and repair the lymphatic injury or ligate the thoracic duct. , Most high-output chylothorax resolves or significantly improves after surgical intervention. For those that do not, an additional week of enteral fasting and octreotide should be attempted before considering pleurodesis or placement of a pleuroperitoneal shunt. , A recent analysis of the Pediatric Health Information Systems (PHIS) database reported that thoracic duct ligation or pleurodesis was performed at a median of 18 days after the cardiac surgery, and patients were discharged from the hospital at a median of 22 days after surgical treatment of chylothorax. More recently, percutaneous thoracic duct embolization has emerged as a less invasive alternative for the treatment of chylothorax. Newer studies, such as dynamic contrast-enhanced magnetic lymphangiography and intranodal lymphangiography, have provided further insight and therapeutic options for this complex disorder. Other individualized supportive therapies include administration of 25% albumin for patients with serum albumin less than 2.5g/dL, intravenous immunoglobulin (IVIG) for those with low IgG levels, and multivitamins.
Separating from mechanical ventilation
Early tracheal extubation of children following congenital heart surgery is not a new concept but has received renewed attention with the evolution of fast-track management for cardiac surgical patients. Early extubation generally refers to tracheal extubation in the operating room or within a few hours (i.e., 4–8 hours) after surgery, although in practice, it means the avoidance of routine overnight mechanical ventilation. Factors to consider when planning early extubation are given in Table 36.2 .
Factor | Consideration |
---|---|
|
|
| |
| |
|
|
| |
| |
| |
|
|
| |
| |
|
|
| |
| |
| |
|
|
| |
|
A number of published reports have described successful tracheal extubation in neonates and older children following congenital heart surgery either in the operating room or soon after in the cardiac ICU. This has been possible without adversely affecting patient care and with a low incidence of reintubation or hemodynamic instability. Such a process can reduce complications such as ventilator-associated events but does not obviate meticulous attention to postoperative analgesia and sedation. The judicious use of this practice has streamlined care and highlights the advances in perioperative care of infants and older children after repair of congenital heart defects.
Separation from mechanical ventilation has the potential to cause important physiologic changes (e.g., increased RV preload, increased LV afterload; see Chapter 32 ); thus, these must be taken into consideration when planning extubation timing. Extubation ideally should occur at the intersect between patient readiness and healthcare team capacity. Although PICUs should strive to provide the same level of care and coverage 24 hours per day every day, it should be recognized that patients with higher complexity and risk often will require a level of undivided attention during and following separation from mechanical ventilation that may compete for attention with concurrent issues affecting other patients in the unit. The decision to extubate ultimately must take these factors into account, and, when necessary, the procedure might benefit from being delayed so that it can be performed under elective conditions and with redundant staffing coverage.
Central nervous system
The dramatic reduction in surgical mortality has been accompanied by a growing recognition of neurologic morbidity in many survivors. In the first months of life, this can manifest in altered tone, abnormal behavior, weak cry, and impaired feeding coordination. Later, these deficits are manifested by cognitive and speech and language dysfunction, impaired visual-motor coordination, learning disorders, and problems with executive functioning. All of these contribute to decreased quality of life and increased cost to society. Neurologic outcomes in patients with critical CHD appear to be multifactorial, involving the interplay of genetic, prenatal, perioperative, and postoperative factors. Treatment in the ICU can impact a number of these factors. Prenatally, the intrauterine circulation for many critical cardiac lesions results in the delivery of less oxygenated blood to the brain, which alters growth and cerebral vascular resistance. The brains of many children with critical CHD demonstrate greater immaturity on brain MRI and have a higher incidence of periventricular leukomalacia (PVL). PVL, much like that seen in premature infants, is associated with increased vulnerability of immature oligodendrocytes to hypoxia and ischemia. Indeed, preoperative hypoxia and diastolic hypotension and postoperative hypotension are all associated with a greater degree of postoperative PVL. Intraoperatively, a number of support techniques used during neonatal and infant cardiac surgery (e.g., CPB, profound hypothermia, circulatory arrest) have been implicated as potential causes of brain injury. These include (1) the total duration of CPB, (2) extreme hemodilution during CPB to hematocrits less than 20, (3) the duration and rate of core cooling, (4) pH management during core cooling, (5) duration of circulatory arrest, (6) position and function of cannulae, and (7) depth of hypothermia. However, the impact of each of these factors is not consistently seen, suggesting the multifactorial nature of CNS injury following CPB. In the postoperative period, the primary factor that most consistently impacts neurodevelopmental outcomes is duration of hospital stay. LOS not only serves as a surrogate for complexity, it also correlates with greater number of medical errors, increased parental stress, and the development of additional morbidities. LOS is also greatly impacted by sedation, prolonged ventilation, and the presence of delirium.
Infants and children undergoing cardiac surgery will require analgesia, sedation, and sometimes paralysis to manage pain, anxiety, oxygen delivery, and hemodynamic instability. However, such agents must be optimally chosen and titrated to avoid under- and overtreatment. Undertreatment can result in an increased stress response, with hemodynamic instability, delayed healing, and the development of posttraumatic stress response. Overtreatment can lead to hypotension, prolonged mechanical ventilation, tolerance, withdrawal, and delayed recovery. This balance can be challenging in infants and young children and those on mechanical ventilation who are unable to communicate. However, the use of validated pain and sedation scores for intubated and nonintubated infants and children that use clinical signs—such as alertness, agitation, muscle tone, facial expression, and response—has been shown to provide more objective measures of adequate pain and sedation management. The use of such scoring systems allows one to tailor therapy to effectively treat pain and minimize oxygen consumption in the most critically ill while also facilitating appropriate state control, early extubation, early mobility, and increased parental involvement. The necessity of more targeted therapy has become increasingly evident with the growing knowledge of the impact of anesthetics, sedatives, and narcotics on the development of delirium and neurologic dysfunction in critically ill infants and children.
Delirium has been increasingly recognized within pediatrics as a driver of prolonged LOS and has been associated with increased mortality and neurologic dysfunction. It is thought to be the consequence of underlying medical illness combined with unwanted side effects of treatment and the stressful environment of the ICU. Possible mechanisms include neuronal injury due to inflammation, microemboli, and global or cellular hypoxia, all of which can be further exacerbated in the presence of critical illness and cardiac surgery with prolonged cardiopulmonary bypass. The incidence of delirium in the pediatric intensive care population has been estimated to be as high as 30%. Those children who are younger than 2 years, require mechanical ventilation, receive benzodiazepines, or require mechanical restraints are at highest risk. In the general critically ill pediatric population, those patients with increased LOS (8 days vs. 4 days) are at greater risk, as are those with inflammatory-mediated disease processes. This may explain the higher incidence and earlier onset of delirium that is seen in the postcardiac surgery population as well as the increased association with duration of CPB.
This suggests that the best approach for a postoperative cardiac patient is to:
- 1.
Routinely assess pain, sedation, and delirium using validated scoring systems to more effectively target therapy. ,
- 2.
Minimize narcotic and sedative use. This can be achieved by the use of a standardized method of treatment of pain that routinely uses scheduled, nonopioid analgesics such as acetaminophen or nonsteroidal antiinflammatory drugs in the immediate postoperative period together with opioid agents. Additionally, the use of agents such as dexmedetomidine, a highly selective α 2 agonist that provides both sedation and analgesia, may have a narcotic and benzodiazepine-sparing effect. Further, dexmedetomidine has been demonstrated to have neuroprotective effects, though the mechanisms remain unclear. While its effect on decreasing heart rate can limit its use, it also has been shown to prevent and treat perioperative arrhythmias.
- 3.
Optimize the environment by minimizing stressful factors and augmenting parental involvement. Cycling of lights, controlling extraneous or excess noise, promoting healthy and consistent sleep, and encouraging parental presence and involvement can both decrease the need for sedation and minimize the development of delirium.
Renal function and postoperative fluid management
Risk factors for postoperative renal failure include preoperative renal dysfunction, prolonged bypass time, hemolysis, low cardiac output, and cardiac arrest. In addition to relative ischemia and nonpulsatile blood flow on CPB, angiotensin II–mediated renal vasoconstriction and delayed healing of renal tubular epithelium have been proposed as mechanisms for renal failure. Postoperative sepsis and nephrotoxic drugs may further contribute to injury.
Serum creatinine is the most widely used test and the current gold standard for diagnosing acute kidney injury (AKI). Using creatinine measurements to diagnose AKI in children has several shortcomings, including—but not limited to—variable normal levels based on age, gender, race, muscle mass, volume status, comorbidities, and use of certain medications. In addition, creatinine assesses only glomerular filtration and functional changes, and levels typically have a delayed rise over days after more than 50% of kidney function is lost in AKI, making it a poor gold standard. These limitations have provided the impetus to search for new biomarkers for the early detection of AKI prior to the functional change heralded by an increase in serum creatinine. Promising new biomarkers include neutrophil gelatinase-associated lipocalin (NGAL), kidney injury molecule-1 (KIM-1), cystatin C, urinary interleukin-18 (IL-18), liver-type fatty acid-binding protein (L-FABP), cell cycle marker insulin-like growth factor binding protein 7 (IGFBP7) and tissue inhibitor of metalloproteinases-2 (TIMP-2). A landmark study of 71 children undergoing CPB showed that the concentrations in serum and urine of NGAL were sensitive, specific, and highly predictive of early AKI after cardiac surgery. In another prospective uncontrolled cohort study, plasma NGAL was shown to be an early predictive biomarker of AKI, morbidity, and mortality after pediatric CPB.
Because of the inflammatory response to bypass and significant increase in total body water, judicious fluid management in the immediate postoperative period is critical. Capillary leak and interstitial fluid accumulation may continue for the first 24 to 48 hours following surgery, necessitating ongoing intravascular volume replacement with colloid or blood products. A fall in cardiac output and increased antidiuretic hormone secretion contribute to delayed water clearance and potential prerenal dysfunction, which could progress to acute tubular necrosis and renal failure if a low–cardiac output state persists.
During CPB, optimizing the circuit prime, hematocrit, and oncotic pressure; attenuating the inflammatory response with steroids; and use of modified ultrafiltration techniques have been recommended to limit interstitial fluid accumulation. During the first 24 hours following surgery, fluids should be restricted to 50% to 66% of full predicted maintenance and volume replacement titrated to appropriate filling pressures and hemodynamic response. Oliguria in the first 24 hours after complex surgery under CPB is common until cardiac output recovers and neurohumoral mechanisms abate. Although diuretics are commonly prescribed in the immediate postoperative period, neurohumoral influences on urine output are powerful and often limit diuretic response. Time after CPB and enhancement of cardiac output through volume and pharmacologic adjustments are the most important factors that will promote diuresis.
Peritoneal dialysis, hemodialysis, and continuous venovenous hemofiltration provide alternate renal support in patients with severe oliguria and AKI. Besides enabling water and solute clearance, maintenance fluids can be increased to ensure adequate nutrition. The indications for renal support vary but include pronounced uremia, life-threatening electrolyte imbalance (such as severe hyperkalemia), ongoing metabolic acidosis, fluid restrictions limiting nutrition, and increased mechanical ventilation requirements secondary to persistent pulmonary edema or ascites.
A peritoneal dialysis catheter may be placed preemptively at the completion of surgery for selected cases or as a bedside procedure later in the ICU, when necessary. Indications include the need for renal support or for reducing intraabdominal pressure from ascites that may compromise mechanical ventilation and splanchnic perfusion. Drainage may be voluminous in the immediate postoperative period as third space fluid losses continue. Replacement of these losses with albumin or FFP may be necessary to treat hypovolemia and hypoproteinemia.
Gastrointestinal issues
Adequate nutrition is important following cardiac surgery in neonates and children. These patients often have decreased caloric intake and increased energy demand after surgery; the neonate, in particular, has limited metabolic and fat reserves. Total parenteral nutrition can provide adequate nutrition in the hypercatabolic phase of the early postoperative period. However, achieving proper caloric intake may be challenging in critically ill patients for whom limited fluid intake and an aggressive fluid removal strategy are a priority (e.g., to facilitate chest closure). For these patients, delaying initiation of parenteral nutrition might be advantageous.
Gastritis, ulcer formation, and upper gastrointestinal bleeding may occur following the stress of cardiac surgery in children and adults. There are limited reports of the efficacy of proton pump inhibitors, histamine H 2 receptor blockers, sucralfate, or oral antacids in pediatric cardiac patients, although their use is common in most PICUs. Hepatic failure may occur after cardiac surgery, particularly after the Fontan operation, and typically is characterized by elevated liver enzymes, hyperammonemia, and coagulopathy.
Necrotizing enterocolitis, although typically a disease of premature infants, is seen with increased frequency in neonates with CHD. Risk factors include (1) left-sided obstructive lesions, (2) umbilical or femoral arterial catheterization/angiography, (3) hypoxemia, and (4) lesions with wide pulse pressures (e.g., systemic-to-pulmonary shunts, severe aortic regurgitation) resulting in diastolic runoff in the mesenteric vessels. Frequently, multiple risk factors exist in the same patient, making a specific etiology difficult to establish. Treatment includes intestinal decompression through continuous nasogastric suction, parenteral nutrition, and broad-spectrum antibiotics. Bowel exploration or resection may be necessary in severe cases with impending or established perforation.
Infection
Low-grade (<38.5°C) fever is common during the immediate postoperative period and may be present for up to 3 to 4 days, even without a demonstrable infectious etiology. However, one ought not to simply disregard the occurrence of fever in the days following surgery, as it might signal an infection, especially in the multiply-instrumented patient. CPB activates complement and other inflammatory mediators but also can lead to derangements of the immune system that increase the likelihood of infection.
Sepsis and nosocomial infection after cardiac surgery contribute substantially to overall morbidity. Despite the increased use of broad antibiotic coverage with third-generation cephalosporins, these agents do not seem to be more effective in decreasing postoperative infections. Most centers use prophylactic coverage with a first-generation cephalosporin (i.e., cefazolin) with the first dose administered in the operating room and continued for the first 24 hours. Type and duration of prophylactic antibiotic coverage may be altered depending on contributing factors (e.g., chest reexploration, transthoracic ECMO cannulation, delayed sternal closure), but these decisions are best made as part of clinical protocols and a robust antibiotic stewardship program to decrease variability and minimize unnecessary exposure. Meticulous catheter insertion and daily care routines, along with early removal of indwelling catheters in the postoperative patient, are important in reducing the incidence of sepsis. Optimal head positioning, mouth care, sedation management, and consideration of an early-extubation strategy can reduce the rates of ventilator-associated events.
Mediastinitis occurs in up to 2% of patients undergoing cardiac surgery. Risk factors include delayed sternal closure, particularly beyond 6 days, early reexploration for bleeding, or reoperation. Mediastinitis is characterized by persistent fever, redness, dehiscence, and purulent drainage from the sternotomy wound, instability of the sternum, and leukocytosis. Staphylococcus is the most common offending organism. Treatment usually involves debridement and irrigation, along with parenteral antibiotic therapy. The duration of therapy depends on the organism and severity of the infection and is generally between 2 and 4 weeks.
Hyperglycemia
Hyperglycemia is a frequent occurrence in the PICU. , As many as 97% and 78% of patients exhibit at least one blood glucose measurement above 125 mg/dL and 200 mg/dL, respectively, following surgical repair of congenital cardiac defects. , The duration of postoperative hyperglycemia in these patients has been strongly and independently correlated with increased morbidity and mortality rates. , Correlation does not signify causation; however, strict glycemic control with insulin administration has been shown to reduce morbidity and mortality rates significantly for adult patients admitted to a surgical ICU, and in one small pediatric study, two large randomized controlled pediatric trials of glycemic control failed to show improvement in meaningful primary outcomes (number of days alive and free from mechanical ventilation, rate of healthcare-associated infection ). In addition, patients assigned to strict glycemic control targeting fasting euglycemia experienced a significant increase in the occurrence of iatrogenic hypoglycemia, , which is just as deleterious, if not more so, than hyperglycemia. , Therefore, strict glycemic control with insulin infusion aimed at fasting euglycemic targets cannot be routinely recommended following cardiac surgery. It may be reasonable to administer an insulin infusion to address severe and persistent postoperative hyperglycemia while targeting the more permissive range, such as the one used in the control arm of the pediatric glycemic trials (150–180 mg/dL).
Critical care management of selected specific lesions
Single-ventricle anatomy and physiology
For a variety of anatomic lesions, the pulmonary and systemic circulations are in parallel with complete mixing, with a single ventricle effectively supplying both systemic and pulmonary blood flow. The proportion of ventricular output to either the pulmonary or systemic vascular bed is determined by the relative resistance to flow in the two circuits. The pulmonary arterial and aortic oxygen saturations are equal. Assuming equal mixing, normal cardiac output, and full pulmonary venous saturation, Sa o 2 of 80% to 85%, with MV o 2 of 60% to 65%, indicates Qp/Qs ≈1 and, hence, a balance between systemic and pulmonary flow. Although “balanced,” the single ventricle still must receive and eject twice the normal amount of blood: one part to the pulmonary circulation and one part to the systemic circulation. A Qp/Qs greater than 1 implies a volume burden on the heart that may have a clinical impact depending on the degree, duration, and myocardial reserve. Though lesion-specific considerations are important in the various types of single-ventricle physiology, common management principles to balance flow and augment systemic perfusion do apply.
Neonatal preoperative management
Changes in PVR have a significant impact on systemic perfusion and circulatory stability, especially preoperatively when the ductus arteriosus is widely patent. In preparation for surgery, it is important that systemic and pulmonary blood flow be as well balanced as possible, especially in the patient who may have accompanying systemic ventricular dysfunction. For example, a newborn with HLHS who has an arterial oxygen saturation greater than 90%, a wide pulse pressure, oliguria, cool extremities, hepatomegaly, and metabolic acidosis has severely limited systemic blood flow. Even though ventricular output is increased, the blood flow that is inefficiently partitioned back to the lungs is unavailable to the other vital organs. Immediate interventions are necessary to prevent imminent circulatory collapse and end-organ injury. In this “overcirculated” state, PVR is falling as it should in the normal postnatal state, and the ductus arteriosus is maintained widely patent to mitigate outflow obstruction from the RV to the systemic circulation. Blood flow manipulation by mechanical ventilation and inotropic support may temporarily stabilize the patient; this should accelerate the timeline for surgical intervention.
Similarly, in a patient with pulmonary atresia and an intact ventricular septum, LV-dependent pulmonary circulation occurs. Ductal patency is necessary for pulmonary blood flow. As PVR falls, pulmonary blood flow will be excessive and eventually will steal from the systemic circulation. Preoperative management should focus on the adequacy of systemic oxygen delivery. This is best achieved by thorough and continuous reevaluation of the clinical examination for cardiac output state and perfusion; evaluation of chest radiograph for cardiac size and pulmonary congestion; review of laboratory data for alterations in gas exchange, acid-base status, and end-organ function; and echocardiographic imaging to assess ventricular function and AV valve competence. In a patient with a good systemic ventricular function, even high pulmonary blood flow (as manifested by higher saturations) is well tolerated for a few days.
However, in the patient without good systemic ventricular function, an assessment of the balance between pulmonary (Qp) and systemic flow (Qs) becomes important. Qp/Qs is equal to the systemic arteriovenous saturation difference (systemic saturation – central venous saturation) divided by the pulmonary venoarterial saturation difference (pulmonary venous saturation [usually estimated] – pulmonary arterial saturation). In all single-ventricle physiologies, the systemic arterial saturations and pulmonary arterial saturations are equal by definition. If this ratio is greater than 2:1 in a patient with clinical or biochemical evidence of insufficient oxygen delivery, additional interventions and/or surgery is necessary.
Initial resuscitation involves maintaining patency of the ductus arteriosus with a PGE 1 infusion at a rate of 0.01 to 0.05 µg/kg per minute. Intubation and mechanical ventilation are not necessary for all patients. Patients usually are tachypneic, but provided that the work of breathing is not excessive and systemic perfusion is maintained without metabolic acidosis, spontaneous ventilation is often preferable in order to achieve adequate systemic perfusion and balance of Qp and Qs. If the initial presentation involved circulatory collapse and end-organ dysfunction, then a period of days may be required to establish stability and allow for the return of vital organ function prior to surgery.
Patients may require intubation and mechanical ventilation because of apnea secondary to PGE 1 , presence of a low–cardiac output state, or for manipulation of gas exchange to assist balancing Qp/Qs. An Sa o 2 greater than 90% indicates pulmonary overcirculation—that is, Qp/Qs greater than 1. PVR can be increased with controlled mechanical hypoventilation to induce respiratory acidosis, often necessitating sedation and neuromuscular blockade, and with a minimization of Fi o 2 to avoid hyperoxic pulmonary vasodilation. Although these maneuvers often are successful in maintaining a relatively high PVR and reducing pulmonary blood flow, it is important to remember that these patients have a limited oxygen reserve and may desaturate suddenly and precipitously. Controlled hypoventilation reduces functional residual capacity (FRC) and, therefore, also decreases the oxygen reserve. Patients who have continued pulmonary overcirculation with high Sa o 2 and reduced systemic perfusion despite these maneuvers require early surgical intervention to control pulmonary blood flow. At the time of surgery, a temporary snare can be placed around either branch of the pulmonary artery to limit pulmonary blood flow effectively. Alternatively, if there are important comorbidities that preclude a standard palliative operation (prematurity, intracranial hemorrhage), bilateral pulmonary arterial bands can be placed through a median sternotomy to optimize systemic blood flow.
Decreased pulmonary blood flow in preoperative patients with a parallel circulation is reflected by hypoxemia with Sa o 2 less than 75%. This may result from restricted flow across a small ductus arteriosus, increased PVR secondary to parenchymal lung disease, or increased pulmonary venous pressure secondary to obstructed pulmonary venous drainage or a restrictive atrial septal defect (ASD). In patients with a later postnatal presentation, blood flow through a restrictive ductus may be augmented by the administration of high-dose PGE 1 (0.1–0.2 µg/kg per minute). Patients at this level of prostaglandin delivery should have their airway secured and may benefit from vasopressor therapy to both offset the vasodilating effects of PGE 1 and increase the systemic vascular resistance (SVR) to augment pulmonary blood flow. Sedation, paralysis, and optimization of mechanical ventilation to maintain an alkalosis may be effective if PVR is elevated. Inhaled nitric oxide (iNO) also may be useful in selected cases. Systemic oxygen delivery can be optimized by augmenting cardiac output and increasing hematocrit level greater than 40%. Among some newborns with HLHS, pulmonary blood flow may be insufficient because mitral valve hypoplasia, in combination with a restrictive or nearly intact atrial septum, severely restricts pulmonary venous return to the heart. The newborn is intensely cyanotic and may have a pulmonary venous congestion pattern on chest radiograph. Urgent interventional cardiac catheterization with balloon septostomy or dilation (or stent placement) of a restrictive ASD may be necessary. , Immediate surgical intervention and palliation are preferred in some centers. Increasingly, these patients are being identified prenatally with an option of fetal catheter-based intervention. Despite such advances, survival in this subgroup is reduced (between 48% and 69%).
Systemic perfusion is maintained with the use of volume and vasoactive agents. Inotropic support is occasionally necessary because of ventricular dysfunction secondary to the increased volume load. This may be of particular concern in the neonate who presents as a postnatal diagnosis. Systemic afterload reduction with agents such as phosphodiesterase inhibitors may improve systemic perfusion, although the reduction in systemic vascular resistance may worsen hypoxemia if this is the primary problem. Oliguria and a rising serum creatinine level may reflect renal insufficiency from a low cardiac output. Necrotizing enterocolitis is a risk secondary to splanchnic hypoperfusion; we prefer not to enterally feed newborns with a wide pulse width and low diastolic pressure (usually <30 mm Hg) prior to surgery. It is important to evaluate end-organ perfusion and function continuously.
Postoperative management
The postoperative management of patients with single-ventricle anatomy and physiology will be discussed later in this chapter, in the section detailing postoperative care of newborns with HLHS following stage I palliation.
Bidirectional cavopulmonary anastomosis
In this procedure, also known as a bidirectional Glenn (BDG) shunt, the SVC is transected and connected end-to-side to the right pulmonary artery, while the pulmonary arteries remain in continuity. Therefore, flow from the SVC is bidirectional into both left and right pulmonary arteries. In most situations, the SVC becomes the only source of pulmonary blood flow, and inferior vena cava (IVC) blood returns to the common atrium. Performed between age 3 to 6 months, the BDG has proved to be an important early staging procedure in single-ventricle physiology for relieving volume and pressure overload, pulmonary artery distortion, and coronary hypoperfusion associated with an aortopulmonary shunt. However, the BDG circulation is not a stable source of pulmonary blood flow in the first few months of life when the PVR is too high to accommodate sufficient passive pulmonary blood flow for tolerable oxygenation. The BDG usually is performed on CPB using mild hypothermia with a beating heart. Therefore, the complications related to CPB and aortic cross-clamping are minimal, and patients can be weaned and extubated in the early postoperative period. In selected cases, the BDG anastomosis can be accomplished without CPB.
Systemic hypertension is common following a BDG. The etiology remains to be determined, but possible factors include improved contractility and stroke volume after the volume load on the ventricle is reduced, and brainstem-mediated mechanisms secondary to the increased systemic and cerebral venous pressure. Treatment with vasodilators may be necessary during the early postoperative period.
Following the BDG anastomosis, arterial oxygen saturation should be in the 80% to 85% range; however, stabilization to this level can take a number of days. In addition, positive-pressure ventilation in these patients reduces passive pulmonary blood flow; thus, these patients are generally excellent candidates for early extubation. Oxygen saturation frequently improves after extubation. Persistent hypoxemia (Sa o 2 <70%) can be secondary to a low–cardiac output state (low mixed venous oxygen saturation [Sv o 2 ]), low pulmonary blood flow, or lung disease ( Table 36.3 ). Treatment is directed at improving contractility, reducing afterload, and ensuring that the patient has a normal rhythm and hematocrit. Increased PVR is an uncommon cause, and iNO is rarely beneficial in these patients. This finding is not surprising because PA pressure and resistance and vascular tone are not high enough following this surgery to see a demonstrable benefit from iNO. Persistent profound hypoxemia should be investigated in the catheterization laboratory to evaluate hemodynamics, to look for residual anatomic defects that might limit pulmonary flow, such as SVC or PA stenosis or a restrictive ASD, and to coil any significant venous decompressing collaterals if present (e.g., SVC to azygous vein).
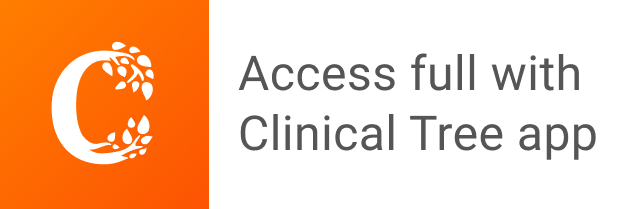