6 Coronary Physiology and Atherosclerosis
Anatomy and Physiology of Blood Vessels
The coronary vasculature has been traditionally divided into three functional groups: large conductance vessels visible on coronary angiography, which offer little resistance to blood flow; small resistance vessels ranging in size from about 10 to 250 μm in diameter; and veins. Although it has been taught that arterioles (precapillary vessels < 50 μm) account for most of the coronary resistance, recent studies indicate that, under resting conditions, 45% to 50% of total coronary vascular resistance resides in vessels larger than 100 μm in diameter1–3 (Figure 6-1). This may be due, in part, to the relatively great length of the small arteries. During intense pharmacologic dilation, the proportion of total coronary vascular resistance because of larger arteries and veins is even greater.2 The regulation of tone in coronary arteries larger than 100 μm in diameter plays an important role in delivering adequate myocardial perfusion.4 One of the early changes in CAD is a diminished ability of the endothelium of epicardial coronary arteries to dilate in response to increased flow (see Endothelium-Derived Relaxing Factors later in this chapter). Advances in technology have enabled measurement, in the beating heart, of diameters of coronary vessels as small as 15 μm. It is becoming evident that, in response to a given intervention, different size classes of coronary vessels can change diameter with different intensity or even in opposite directions.5,6 This heterogeneity of response according to vessel size would be an important consideration in predicting the effects of vasoactive agents on myocardial perfusion. For example, a drug that dilated large vessels and collaterals but not arterioles would be beneficial to patients with CAD (see Coronary Steal later in this chapter).

Normal Artery Wall
The arterial lumen is lined by a monolayer of endothelial cells that overlies smooth muscle cells (Figure 6-2). The inner layer of smooth muscle cells, known as the intima, is circumscribed by the internal elastic lamina. Between the internal elastic lamina and external elastic lamina is another layer of smooth muscle cells, the media. Outside the external elastic lamina is an adventitia that is sparsely populated by cells and microvessels of the vasa vasorum.

Intima
Traditionally, the intima has been considered the most important layer of the artery wall.7 The intima can vary from a single endothelial layer to a more complex structure of an endothelium overlying a patchwork of extracellular matrix and vascular smooth muscle cells. As part of the normal development of many large arteries, smooth muscle cells populate this space and form a neointima. This diffuse form of intimal thickening consists of layers of smooth muscle cells and connective tissue, the thickness of which may vary considerably. For convenience, the intima/media ratio is often measured, and the reference range is 0.1 to 1.0. How this benign intima forms is not well understood. Presumably, the intima represents a physiologic adaptation to changes in arterial flow and wall tension. The intima is made up of two distinct layers.8 As seen by electron microscopy, the inner layer subjacent to the luminal endothelium contains an abundance of proteoglycan ground substance. Smooth muscle cells found in this layer are usually distributed as isolated cells in a sea of matrix, rather than in contiguous layers. A few macrophages also may be found in this layer underneath the endothelial monolayer. The outer, musculoelastic layer of the intima is adjacent to the internal elastic lamina and contains smooth muscle cells and elastic fibers.

Media
In normal adult arteries, several smooth muscle cell subpopulations with distinct lineages exist within the media.9 These diverse cell populations likely fulfill different functions to maintain homeostasis in the artery wall. For example, in response to pressure elevations, increases in smooth muscle cell mass and extracellular matrix may be required. Alternatively, for arteries to be able to stretch both longitudinally and circumferentially, smooth muscle cells with variable orientations of cytoskeletal fibers must be present. These distinct cell types may be important not only in health but in disease. In certain experimental models of neointimal formation, proliferation and inward migration of subpopulations of medial smooth muscle cells occur.10 The biologic determinants of medial smooth muscle cell diversity are unknown.11

Adventitia
The adventitia, the outermost layer of the artery wall, normally consists of a sparse collection of fibroblasts, microvessels (vasa vasorum), nerves, and few inflammatory cells. The majority of the vasa vasorum that nourish the inner layers of the artery wall originate in the adventitia. Traditionally, the adventitia has been ignored and is not thought to play a role in vascular lesion formation. However, more recent studies have elucidated the role of the adventitia as not only a source of inflammatory cells in the development of atherosclerosis, but a hub for paracrine signaling that can maintain vascular homeostasis in a variety of vascular diseases. 12

Transmembrane and Transcellular Communication
Figure 6-3 illustrates examples of pathways of transmembrane signaling. Up to five components can be involved: receptor, G protein, effector producing a second messenger, phosphorylation of regulator protein, and the consequent change in cell behavior. G proteins (guanine nucleotide-binding regulatory proteins) are made up of three subunits (α, β, γ) and float in the cell membrane. On contact with a ligand-receptor complex, guanosine diphosphate (GDP) on the α subunit is replaced by guanosine triphosphate (GTP). The activated α subunit then dissociates from the beta-gamma complex and can interact with several membrane targets (see Figure 6-3B). For example, β-receptor activation results in the activation of Gs (s = stimulate), which will stimulate the synthesis of cAMP by adenylyl cyclase. Muscarinic receptor activation activates a Gi (i = inhibit) protein that inhibits adenylyl cyclase. A single G protein can interact with more than one effector. In this way, the G protein can be a branch point for the regulation of multiple effectors in response to a single signal. These proteins already have been implicated in human disease; cholera toxin covalently modifies Gs so that it becomes persistently active in stimulating adenylyl cyclase in intestinal epithelial cells, likely causing the severe diarrhea of cholera.

Figure 6-3 Steps in the process whereby hormone-receptor binding results in a change in cell behavior.
(A, B, From Brown AM, Birnbaumer L: Ionic channels and their regulation by G-protein subunits. Annu Rev Physiol 52:197, 1990.)
Several second-messenger systems have been characterized. Gs can directly enhance conductance through calcium channels, with the increased intracellular calcium acting as second messenger. The cyclic nucleotides, cAMP and guanosine monophosphate (GMP), act as second messengers. Their intracellular action is terminated when they are cleaved by phosphodiesterase enzymes, which, in turn, are regulated by stimuli and second messengers. The breakdown products of membrane phosphoinositide constitute another, more recently recognized set of second messengers.13 In response to agonists such as vasopressin, G protein is activated, leading to activation of the membrane-associated enzyme phospholipase C. This enzyme cleaves phosphatidylinositol 4,5-biphosphate on the inner leaflet of the plasma membrane, producing inositol 1,4,5-triphosphate (IP3) and diacylglycerol (DAG). Both are second messengers. IP3 diffuses through the cytoplasm and mobilizes calcium from intracellular stores. DAG remains within the plasma membrane and activates protein kinase C, which modulates cellular activity by phosphorylating intracellular proteins. In many cell types, activation of the same receptors that control phosphoinositide breakdown also results in the liberation of arachidonate and/or eicosanoids (prostaglandins, leukotrienes, and thromboxanes). The resultant change in cell behavior can be the opening of an ion channel, contraction or relaxation of smooth muscle, secretory activity, or initiation of cell division (see Chapter 7).

Endothelium
Although the vascular endothelium was once thought of as an inert lining for blood vessels, it is more accurately characterized as a very active, distributed organ with many biologic functions. It has synthetic (Table 6-1) and metabolic (Table 6-2) capabilities, and contains receptors for a variety of vasoactive substances (Table 6-3). Functions of the endothelium that may play an important role in the pathophysiology of ischemic heart disease are discussed.
TABLE 6-1 Substances Produced by Vascular Endothelium
Antithrombotic Substances | Procoagulants |
---|---|
Prostacyclin | von Willebrand factor |
Antithrombin III | Collagen |
Plasminogen activator | Fibronectin |
Protein C | Thromboplastin |
α2-Macroglobulin | Thrombospondin |
Glycosaminoglycans (heparin) | Plasminogen inhibitors |
Platelet-activating factor | |
Thromboxane A2 |
From Bassenge E, Busse R: Endothelial modulation of coronary tone. Prog Cardiovasc Dis 30:349, 1988.
TABLE 6-2 Vasoactive Substances Processed by Vascular Endothelium
Uptake and Metabolism | Enzymatic Conversion or Degradation |
---|---|
Norepinephrine | Angiotensin I to angiotensin II (ACE) |
Serotonin | Angiotensin II to angiotensin III (angiotensinase) |
Prostaglandins (E1, E2, E2α) | Bradykinin degradation (ACE) |
Leukotrienes | Substance P degradation |
Adenosine |
ACE, angiotensin-converting enzyme.
From Bassenge E, Busse R: Endothelial modulation of coronary tone. Prog Cardiovasc Dis 30:349, 1988.
TABLE 6-3 Stimulators of Endothelium-Mediated Vasodilation
Transmitters | Adenosine diphosphate (ADP) |
Acetylcholine | Adenosine |
Norepinephrine | Serotonin |
Peptides | Thrombin |
Angiotensin | Trypsin |
Bradykinin | Local Hormones |
Vasopressin | Histamine |
Oxytocin | Platelet-activating factor |
Substance P | Physicochemical Stimuli |
Vasoactive intestinal peptide | Shear stress (flow) |
Calcitonin gene–related peptide | Mechanical stress (pulsatility) |
Platelet or Blood Components | Hypoxia |
Adenosine triphosphate (ATP) |
Modified from Bassenge E, Busse R: Endothelial modulation of coronary tone. Prog Cardiovasc Dis 30:349, 1988.
Endothelium-Derived Relaxing Factors
The first vasoactive endothelial substance to be discovered was prostacyclin (PGI2), a product of the cyclooxygenase pathway of arachidonic acid metabolism (Figure 6-4; Box 6-1).14 The production of PGI2 is activated by shear stress, pulsatility of flow, hypoxia, and a variety of vasoactive mediators. On production it leaves the endothelial cell and acts in the local environment to cause relaxation of the underlying smooth muscle or to inhibit platelet aggregation. Both actions are mediated by the stimulation of adenylyl cyclase in the target cell to produce cAMP.

Figure 6-4 The production of endothelium-derived vasodilator substances.
(From Rubanyi GM: Endothelium, platelets, and coronary vasospasm. Coron Artery Dis 1:645, 1990.)
In 1980, Furchgott and Zawadzki15 observed that the presence of an intact endothelium was necessary for acetylcholine-induced vasodilation. Since that time it has been shown that many physiologic stimuli cause vasodilation by stimulating the release of a labile, diffusible, nonprostanoid molecule termed endothelium-derived relaxing factor (EDRF; see Figure 6-4), now known to be nitric oxide (NO). NO is the basis of a widespread paracrine signal transduction mechanism whereby one cell type can modulate the behavior of adjacent cells of different type.16,17 NO is a very small lipophilic molecule that can readily diffuse across biologic membranes and into the cytosol of nearby cells. The half-life of the molecule is less than 5 seconds, so that only the local environment can be affected. NO is synthesized from the amino acid l-arginine by nitric oxide synthase (NOS). In vascular endothelium, the enzyme (endothelial NOS or NOS3) is always present (constitutive) and resides in the cytoplasm. Its function depends on the presence of Ca++ and calmodulin, as well as tetrahydrobiopterin. Serine phosphorylation is important for prolonged activity. The enzyme is activated in response to receptor occupancy or physical stimulation (see Table 6-3). When NO diffuses into the cytosol of the target cell, it binds with the heme group of soluble guanylate cyclase, resulting in a 50- to 200-fold increase in production of cyclic GMP, its second messenger. If the target cells are vascular smooth muscle cells, vasodilation occurs; if the target cells are platelets, adhesion and aggregation are inhibited. In vascular smooth muscle, cyclic GMP leads to activation of protein kinase G, which phosphorylates various intracellular target proteins, including the myosin light-chain regulatory subunit and proteins that control intracellular calcium.18
It is likely that NO is the final common effector molecule of nitrovasodilators (including sodium nitroprusside and organic nitrates such as nitroglycerin). The cardiovascular system is in a constant state of active vasodilation that is dependent on the generation of NO. The molecule is more important in controlling vascular tone in veins and arteries compared with arterioles. When the microcirculation dilates in response to metabolic myocardial demand (e.g., exercise), increased flow through epicardial coronary arteries increases shear stress at the endothelium. This leads to release of NO, which causes vascular smooth muscle relaxation and dilation of the conductance vessels, thereby facilitating the increase in flow. The importance of the loss of this mechanism in atherosclerosis is underlined by the fact that, in this situation, more than 50% of the resistance to flow in the coronary circulation resides in vessels larger than 100 μm in diameter (see Figure 6-1). Abnormalities in the ability of the endothelium to produce NO likely plays a role in diseases such as diabetes, atherosclerosis, and hypertension.19,20 The venous circulation of humans appears to have a lower basal release of NO and an increased sensitivity to nitrovasodilators when compared with the arterial side of the circulation.21
Many agents, such as acetylcholine and norepinephrine, can cause contraction when applied directly to the vascular smooth muscle membrane instead of relaxation, which occurs when it is applied to the intact endothelium (Figure 6-5). The net effect of neural or humoral stimuli depends on a combination of direct effects mediated by binding to vascular smooth muscle receptors and indirect effects because of the ligand binding to endothelial receptors causing NO release from the endothelium. In the presence of healthy endothelium, vasodilation usually predominates. When the endothelium is absent (injured vessel) or diseased (atherosclerosis), vasoconstriction may be the net effect. NO has important roles in neurohumoral regulation of vascular tone, in preventing intravascular platelet aggregation, and in the structural adaptation of blood vessels to the demands of blood flow and pressure. Knowledge of its role in inflammation and atherosclerosis is rapidly expanding.

Figure 6-5 Role of endothelium in the control of coronary tone.
(From Bassenge E, Heusch G: Endothelial and neurohumoral control of coronary blood flow in health and disease. Rev Physiol Biochem Pharmacol 116:77, 1990.)
In addition to PGI2 and NO, another less-well-understood pathway for receptor-mediated or mechanically induced endothelium-derived vasodilation exists that is associated with smooth muscle hyperpolarization. Both epoxyeicosatrienoic acid (a metabolite of cytochrome P450) and H2O2 have been suggested as possible endothelium-derived hyperpolarizing factors (EDHFs).22,23 Smooth muscle relaxation is a result of hyperpolarization of the myocyte, which leads to decreased intracellular calcium concentration. EDHF-mediated vasodilation can be blocked by inhibition of calcium-dependent potassium channels. EDHF may have an important vasodilator role in the human coronary microcirculation.24
Endothelium-Derived Contracting Factors
Contracting factors produced by the endothelium include prostaglandin H2, thromboxane A2 (TxA2; via cyclooxygenase), and the peptide endothelin (ET). ET is a potent vasoconstrictor peptide (100-fold more potent than norepinephrine)25 with remarkable similarities to the toxin of the burrowing asp. Both have potent coronary constrictor activity to which the strong cardiac toxicity and lethality of the toxin are attributed.26 Three closely related 21 amino acid peptides have been identified: endothelin-1 (ET-1), ET-2, and ET-3. The primary product of vascular endothelium is ET-1, which is synthesized from prepro-ET-1 within vascular endothelial cells by the action of ET-converting enzyme. It is not stored but rapidly synthesized in response to stimuli such as ischemia, hypoxia, and shear stress, and released predominantly abluminally (toward the underlying smooth muscle).27 In vascular smooth muscle cells, ET-1 binds to specific membrane receptors (ETA) and, via phospholipase C, induces an increase in intracellular calcium resulting in long-lasting contractions.28 It is also linked via a Gi protein to voltage-operated calcium channels. This peptide has greater vasoconstricting potency than any other cardiovascular hormone, and in pharmacologic doses can abolish coronary flow, leading to ventricular fibrillation and death.29 Another receptor subtype, ETB, is expressed by both smooth muscle and endothelium and binds ET-1 and ET-3 equally well (Figure 6-6). When isolated vessels are perfused with ET-1, there is an initial NO-mediated vasodilation because of binding with ETB receptors on the endothelial cells, followed by contraction because of binding of ET-1 to ETA receptors on the vascular smooth muscle membrane. Studies utilizing bosentan, a combined ETA– and ETB-receptor antagonist, have demonstrated that ET exerts a basal coronary vasoconstrictor tone in humans.30 There is evidence that ET may play a role in the pathophysiology of pulmonary and arterial hypertension, atherosclerosis, myocardial ischemic syndromes, and heart failure.31 Clinical trials of bosentan in patients with congestive heart failure32 and hypertension have shown promise, but hepatic side effects have limited the dose to less than 500 mg daily, with the primary indication being severe pulmonary hypertension.33
Endothelial Inhibition of Platelets
A primary function of endothelium is to maintain the fluidity of blood. This is achieved by the synthesis and release of anticoagulant (e.g., thrombomodulin, protein C), fibrinolytic (e.g., tissue-type plasminogen activator), and platelet inhibitory (e.g., PGI2, NO) substances (Box 6-2).34 Mediators released from aggregating platelets stimulate the release of NO and PGI2 from intact endothelium, which act together to increase blood flow and decrease platelet adhesion and aggregation (Figure 6-7), thereby flushing away microthrombi and maintaining the patency of the vessel.
BOX 6-2 Endothelial Inhibition Of Platelets
Healthy endothelial cells have a role in maintaining the fluidity of blood by producing:

Figure 6-7 Inhibition of platelet adhesion and aggregation by intact endothelium.
(From Rubanyi GM: Endothelium, platelets, and coronary vasospasm. Coron Artery Dis 1:645, 1990.)
With vital roles in modulating the tone of vascular smooth muscle, inhibiting platelets, and processing circulating chemicals, it seems clear that endothelial cell dysfunction would cause or contribute to ischemic syndromes. There is evidence of endothelial dysfunction in atherosclerosis, hyperlipidemia, diabetes, and hypertension.35 Procedures such as coronary artery surgery and angioplasty disrupt the endothelium. The role of endothelium in the pathophysiology of myocardial ischemia is discussed later (see Dynamic Stenosis section).
Determinants of Coronary Blood Flow

Perfusion Pressure and Myocardial Compression
Coronary blood flow is proportional to the pressure gradient across the coronary circulation (Box 6-3). This gradient is calculated by subtracting downstream coronary pressure from the pressure in the root of the aorta. The determination of downstream pressure is complicated because the intramural coronary vessels are compressed with each heartbeat.
During systole, the heart throttles its own blood supply. The force of systolic myocardial compression is greatest in the subendocardial layers, where it approximates intraventricular pressure. Resistance caused by extravascular compression increases with blood pressure, heart rate, contractility, and preload. Because it is difficult to measure intramyocardial pressure, the relative importance of these factors is controversial.36,37 Flow is impeded both by direct compression and by shear caused by twisting of vessels as the heart contracts. Myocardial extravascular compression is less in the right ventricle, where pressures are lower and coronary perfusion persists during systole (Figure 6-8). In pathologic conditions associated with pulmonary hypertension, right coronary flow assumes a phasic pattern similar to left coronary flow. Under normal conditions, extravascular compression contributes only a small component (10% to 25%) to total coronary vascular resistance. When the coronary vessels are dilated by pharmacologic agents such as dipyridamole or during ischemia, the effects of extravascular compression on myocardial perfusion become more important (see Transmural Blood Flow section later in this chapter).

Figure 6-8 Blood flow in the left and right coronary arteries.
(From Berne RM, Levy MN: Special circulations. In Berne RM, Levy MN [eds]: Physiology. St. Louis: CV Mosby, 1988, pp 540–560.)
With each contraction, the intramural vessels are squeezed and blood is expelled forward into the coronary sinus and retrograde into the epicardial arteries. The large coronary arteries on the epicardial surface act as capacitors, charging with blood during systole and expelling blood into the coronary circulation during diastole.38 Coronary capacitance likely explains the findings of Bellamy,39 who reported that flow in the proximal left anterior descending coronary artery of the dog ceased when arterial pressure decreased to less than 45 mm Hg. It was suggested that flow throughout the coronary circulation stopped at pressures far in excess of the pressure at the coronary sinus. This pressure at which flow stopped was termed critical closing pressure or zero-flow pressure (Pzf). This had important implications in the calculation of coronary resistance because the effective downstream pressure would be Pzf and not the much lower coronary venous pressure. This is analogous to a stream with a waterfall, where flow rate over the waterfall depends on the drop from the source to the waterfall edge and is unaffected by the distance to the bottom of the falls. It was later suggested that flow through the intramural coronary vessels continues after coronary inflow near the ostia (measured by Bellamy) has ceased.40,41 There is evidence that antegrade movement of red blood cells in 20-μm arterioles continues until coronary pressure is only a few millimeters of mercury greater than coronary sinus pressure.42 The concept of a critical closing pressure greatly in excess of coronary sinus pressure is probably not valid in the coronary circulation.
Although the true downstream pressure of the coronary circulation is likely close to the coronary sinus pressure, other choices may be more appropriate in clinical circumstances. In patients with CAD, the subendocardial layers of the left ventricle are at greatest risk for ischemia and necrosis (see Transmural Blood Flow section later in this chapter). Because this layer is perfused mostly when the aortic valve is closed, the most appropriate measure of the driving pressure for flow here is the average pressure in the aortic root during diastole. This can be approximated by aortic diastolic or mean pressure. Pressures monitored in peripheral arteries by routine methods in clinical settings can differ from central aortic readings. This is due to distortion of the pressure waveform as it is propagated through the arterial tree and inaccuracies associated with the hydraulic and electronic components of the monitoring system. Under these conditions, the mean arterial pressure may be the most reliable measure of coronary driving pressure. The true downstream pressure of the left ventricular subendocardium is the left ventricular diastolic pressure, which can be estimated by pulmonary artery occlusion pressure. When the right ventricle is at risk for ischemia (e.g., severe pulmonary hypertension), right ventricular diastolic pressure or central venous pressure may be more appropriate choices for downstream pressure.

Myocardial Metabolism
Myocardial blood flow, like flow in the brain and skeletal muscle, is primarily under metabolic control. Even when the heart is cut off from external control mechanisms (neural and humoral factors), its ability to match blood flow to its metabolic requirements is almost unaffected.35 Because coronary venous oxygen tension is normally 15 to 20 mm Hg, there is only a small amount of oxygen available through increased extraction. A major increase in cardiac oxygen consumption (Mvo2) can occur only if oxygen delivery is increased by augmentation of coronary blood flow. Normally, flow and metabolism are closely matched so that over a wide range of oxygen consumption, coronary sinus oxygen saturation changes little.43
Despite intensive research over the last several decades, the mediator or mediators linking myocardial metabolism so effectively to myocardial blood flow are still unknown. Hypotheses of metabolic control propose that vascular tone is linked either to a substrate that is depleted, such as oxygen or adenosine triphosphate (ATP), or to the accumulation of a metabolite such as CO2 or hydrogen ion (Box 6-4). Adenosine has been proposed in both categories. Feigl43 has proposed six criteria for a chemical transmitter between the cardiac myocyte and the coronary vascular smooth muscle cell:
Many potential mediators of metabolic regulation have been proposed.44 Although NO has a role in many coronary vasoregulatory pathways, it does not fulfill the role of metabolic regulator because blockade of NOS does not alter the increase in myocardial blood flow associated with an increase in myocardial oxygen demand.45 The arguments for oxygen, carbon dioxide, and adenosine are briefly examined.
Oxygen
The coronary smooth muscle would have to be more sensitive to lack of oxygen than the working cardiocytes for oxygen to regulate coronary flow through a direct vascular action. Coronary microvessels in vitro do not relax until Po2 is less than 5 mm Hg, a level well below the average Po2 of 20 mm Hg in cardiac muscle cytosol.46,47 With myocardial oxygen consumption (Mvo2) held constant, increases in arterial oxygen content cause coronary flow to decrease, whereas decreases in arterial oxygen content cause flow to increase. These changes could explain only 40% of the increase in flow observed with tachycardia.48 It is undecided whether the constancy of myocardial oxygen tension is the cause or the consequence of the excellent match between myocardial metabolism and myocardial blood flow.49
Carbon Dioxide
The end product of substrate oxidation is CO2, the formation of which is directly related to the level of cardiac work. Carbon dioxide is highly diffusible and can easily reach coronary smooth muscle cells. Unfortunately, it is difficult to separate the effects on coronary tone of increasing CO2 from concomitant increases in other metabolites. Broten et al48 pump-perfused the left main coronary artery of dogs and used an oxygenator in the perfusion circuit to alter coronary arterial Pco2 and Po2 at a constant level of myocardial metabolism. Increases in arterial and coronary sinus Pco2 caused increases in coronary blood flow in the absence of changes in Mvo2. Interestingly, there was a synergistic action of Pco2 and Po2: The increase in flow with elevation of Pco2 was much greater at low Po2 and vice versa. The effect of increasing CO2, however, could not completely account for flow changes associated with an increase in Mvo2.
Adenosine
Adenosine is a powerful coronary vasodilator via its activation of receptors on vascular endothelium and smooth muscle. In 1963, both Berne50 and Gerlach51 independently demonstrated the production of adenosine in ischemic heart muscle. They hypothesized that the release of adenosine may serve as a feedback signal inducing coronary vasodilation and augmenting coronary blood flow in proportion to myocardial metabolic needs. Initially, it was suggested that adenosine formation was coupled to myocardial oxygen tension.50 A substrate theory has been proposed whereby adenosine production is linked to the cardiac energy state by the regulation of cytosolic AMP concentration to explain metabolic regulation by adenosine under both normoxic and ischemic conditions.52 According to this theory, increases in cardiac work lead to a decline in ATP potential, which results in a quantitatively appropriate change in cytosolic AMP concentration, leading to increased adenosine release. In this way, the rate of adenosine production is determined by the myocardial oxygen supply/demand ratio. It is likely that adenosine causes coronary arteriolar dilation through stimulation of A1 receptors directly coupled to ATP-sensitive K+ (K+ATP) channels and A2 receptor–mediated elevation of cAMP/protein kinase A, which lead to vasodilation, in part, by opening of K+ ATP channels.53,54
Evidence against the adenosine hypothesis is accumulating. Adenosine deaminase is an enzyme that, when introduced in sufficient quantity into the myocardium, can significantly reduce the interstitial concentration of adenosine. Aminophylline and theophylline interfere with the coronary dilating effects of adenosine by acting on the receptor on vascular smooth muscle. Experiments using these agents to inhibit adenosine effect have shown that resting coronary blood flow, exercise-induced coronary dilation, autoregulation, and reactive hyperemia are largely unrelated to adenosine.55–58 Measuring coronary microvessel diameters in beating hearts in situ, Kanatsuka et al5 found that when Mvo2 was doubled by pacing, vessels between 40 and 380 μm dilated, whereas when a similar increase in flow was induced by the infusion of adenosine or dipyridamole at constant Mvo2, only vessels smaller than 150 μm dilated. Although adenosine does not seem to have an important role in metabolic regulation in the normal heart, adenosine blockade has been shown to cause a decrease in blood flow to hypoperfused myocardium sufficient to decrease systolic segment shortening.59 Adenosine may have other important roles in ischemia, in which there is evidence of a cardioprotective action.60,61

Neural and Humoral Control
Coronary Innervation
The heart is supplied with branches of the sympathetic and parasympathetic divisions of the autonomic nervous system. Thicker vagal fibers end in the adventitia of coronary vessels, whereas fine nonmedullated sympathetic fibers end on vascular smooth muscle cells.62 Large and small coronary arteries, as well as veins, are richly innervated. The sympathetic nerves to the heart and coronary vessels arise from the superior, middle, and inferior cervical sympathetic ganglia, as well as the first four thoracic ganglia. The stellate ganglion (formed when the inferior cervical and first thoracic ganglia merge) is a major source of cardiac sympathetic innervation. The vagi supply the heart with efferent cholinergic nerves.
Parasympathetic Control
Vagal stimulation causes bradycardia, decreased contractility, and lower blood pressure. The resultant decline in Mvo2 causes a metabolically mediated coronary vasoconstriction. When myocardial metabolism is held constant, however, cholinergic coronary dilation is consistently observed in response to exogenous acetylcholine, electrical vagal stimulation, and reflex activation through baroreceptors, chemoreceptors, and ventricular receptors.35,43,63 These effects can be abolished by atropine.
In patients with angiographically normal coronary arteries, the response to intracoronary acetylcholine injection is predominantly dilation, whereas in atherosclerotic segments of epicardial arteries, constriction is observed.64–66 Acetylcholine injected intraluminally binds to muscarinic receptors on the endothelium and stimulates the release of NO, which causes smooth muscle dilation. Acetylcholine is not normally found circulating in the blood but is released from vagal fibers and reaches the coronary smooth muscle from the adventitial side. Surprisingly, activation of muscarinic receptors on vascular smooth muscle cells causes constriction. Parasympathetic stimulation normally causes coronary vasodilation. This response depends on the ability of the coronary endothelium to elaborate NO and perhaps also EDHF (see earlier).67,68 Parasympathetic control has not been shown to be important in the initiation of myocardial ischemia.
β-Adrenergic Coronary Dilation
β-Receptor activation causes dilation of both large and small coronary vessels even in the absence of changes in blood flow.35,43 Studies in animals indicate that both β1 and β2 receptors are present throughout the coronary circulation, but β1 receptors predominate in the conductance vessels, whereas β2 receptors predominate in the resistance vessels. Mature canine coronary collaterals respond similarly to the conductance vessels.69,70 β-Adrenergic coronary dilation may improve the speed and accuracy of coronary blood flow regulation during exercise.71
α-Adrenergic Coronary Constriction
Activation of the sympathetic nerves to the heart results in increases in heart rate, contractility, and blood pressure, which lead to a marked, metabolically mediated increase in coronary blood flow (Box 6-5). This suggested to early investigators that the effect of sympathetic coronary innervation is vasodilation. More recent investigation has demonstrated that the direct effect of sympathetic stimulation is coronary vasoconstriction, which is in competition with the metabolically mediated dilation of exercise or excitement. Whether adrenergic coronary constriction is powerful enough to further diminish blood flow in ischemic myocardium or whether it can have some beneficial effect in the distribution of myocardial blood flow is controversial.
Classification
α-Adrenergic receptors can be classified anatomically as presynaptic or postsynaptic and also according to their pharmacologic properties as α1 and α2 (Table 6-4). The receptors can be further divided into subtypes according to their signal transduction mechanism (G-protein subtype) and second messenger (adenylyl cyclase, phospholipase C, etc.).72
TABLE 6-4 Classification of α-Adrenergic Receptor Subtypes in the Heart
Selective Agonists | Selective Antagonists | Effects of Activation |
---|---|---|
α1 | ||
Phenylephrine | Prazosin | Presynaptic: feedback inhibition of norepinephrine release |
Methoxamine | Postsynaptic: coronary vasoconstriction, increase in myocardial arrhythmias | |
Inotropism | ||
α2 | ||
Clonidine | Yohimbine | Presynaptic: feedback inhibition of norepinephrine release |
Azepexole | Rauwolscine | Postsynaptic: coronary vasoconstriction, arrhythmias (?) |
BHT 920 | Idazoxan | |
UK 14, 304 |
Norepinephrine is a nonselective agonist. Phentolamine and phenoxybenzamine are nonselective antagonists. Phenylephrine also causes β-receptor activation.
Modified from Heusch G: Alpha-adrenergic mechanisms in myocardial ischemia. Circulation 81:1, 1990.
Presynaptic α Receptors
α receptors on cardiac sympathetic nerve terminals mediate feedback inhibition of neuronal norepinephrine release. Both α1 and α2 receptors appear to be involved because exercise-induced increases in heart rate and contractility can be potentiated by either idazoxan (α2-blockade) or prazosin (α1-blockade).73
Cardiac Muscle Cells
Activation of myocardial α1 receptors results in a positive inotropic effect that, in contrast with β-receptor activation, is associated with prolongation of contraction. Although normally of minor functional importance, this effect may serve as an inotropic reserve mechanism when β-receptor–mediated inotropy is impaired (e.g., hypothyroidism, cardiac failure, chronic propranolol treatment).74 The importance of this mechanism in humans is uncertain. An increase in inotropy caused by stimulation of myocardial α receptors would result in increased Mvo2 and a metabolically mediated coronary dilation.
Coronary Endothelium
Binding of norepinephrine to α2 receptors on vascular endothelium stimulates the release of NO, which acts to relax vascular smooth muscle. The endothelium can also act to limit the effect of norepinephrine by metabolizing it. In these ways, the endothelium modulates the direct constrictive effects of α-adrenergic activation. Abnormal endothelial function in atherosclerosis may predispose to excessive α-adrenergic constriction and is implicated in the pathogenesis of myocardial ischemia (see Dynamic Stenosis later in this chapter).
Coronary Resistance
The magnitude of α-adrenergic vasoconstriction that occurs in the coronary bed is small compared with that which occurs in the skin and skeletal muscle. In the presence of β-blockade, intense sympathetic stimulation results in only a 20% to 30% increase in coronary resistance.75 Mohrman and Feigl76 examined the effect of sympathetic activation on coronary flow in the absence of β-blockade. The net effect of α-receptor vasoconstriction was to restrict the metabolically related flow increase by 30%, thereby increasing oxygen extraction and decreasing coronary sinus oxygen content.
Epicardial coronary diameter changes little during sympathetic stimulation.77 α1-Adrenergic and α2-adrenergic receptors are found throughout the coronary circulation; however, α1 receptors appear to be more important in the large epicardial vessels, whereas α2 predominate in small coronary vessels less than 100 μm in diameter.78 Studies of mature coronary collateral vessels in dogs have generally failed to provide evidence of α-receptor–mediated vasoconstriction.79 After heart transplant, patients demonstrated a lesser increase in myocardial blood flow after a cold pressor test in denervated regions of the heart.80 The authors argue that this was not due to increased myocardial metabolism secondary to myocardial β-receptor activation. They suggest that sympathetic innervation has an important role in coronary vessel dilation during stress.
Exercise
α-Adrenergic coronary constrictor tone during exercise is exerted predominantly by circulating catecholamines.81 Numerous studies indicate that myocardial blood flow during exercise is limited by α vasoconstriction.35 In a study of exercising dogs, Huang and Feigl82 found that despite an increase in total coronary flow in an α-blocked region of myocardium, flow to the inner, subendocardial layer was diminished. These results suggest a beneficial effect of α-adrenergic coronary constriction on the distribution of blood flow within the myocardium.
Myocardial Ischemia
Buffington and Feigl83 demonstrated the persistence of α-adrenergic coronary vasoconstriction distal to a moderate coronary stenosis during norepinephrine infusion. Investigations in dogs have demonstrated that, as coronary reserve is depleted by increasing stenosis severity, the response to sympathetic stimulation shifts from a metabolically induced coronary dilation to coronary constriction.84,85 These observations suggest that sympathetic coronary vasoconstriction limits coronary blood flow even during myocardial ischemia, when autoregulatory reserve is exhausted (see Coronary Reserve later in this chapter). There is no consensus as to the importance of α1 vs α2 receptors in ischemic myocardium.35 Using constant flow coronary perfusion in anesthetized dogs, Nathan and Feigl86 compared the transmural distribution of myocardial blood flow in α-blocked and intact regions of myocardium during hypoperfusion. Surprisingly, α-blockade diverted blood flow from the subendocardium to the subepicardium. This suggests that α vasoconstriction had limited flow more in the subepicardium, thereby producing an antisteal effect, and improved perfusion of the more vulnerable inner layers of the left ventricle. Chilian and Ackell87 found similar results in exercising dogs with an artificial coronary stenosis. In contrast, work from Heusch and colleagues88,89 demonstrated improved subendocardial perfusion distal to a severe coronary stenosis with α2-receptor blockade. This controversy is unresolved.90 α-Receptor blockers have not been shown to have a role in the treatment of myocardial ischemia in patients with CAD.
Studies in Humans
Studies indicate that there is little α-adrenoceptor–mediated tone in resting humans.91 Clinical studies have failed to provide convincing evidence that α-adrenergic coronary constriction plays an important role in Prinzmetal’s variant angina (angina with ST-segment elevation at rest).92 During sympathetic activation, however, there is evidence that α vasoconstriction can precipitate myocardial ischemia by further narrowing diseased coronary arteries. This has been shown during isometric exercise, dynamic exercise, and with the cold pressor test93–98 (see Dynamic Stenosis later in this chapter).
Humoral Control
A complete understanding of the effects of circulating substances on the coronary vessels would require determining their effects on large versus small coronary vessels, while separating direct effects on coronary vessels from changes in tone mediated by changes in myocardial metabolism. This is further complicated by the critical role of an intact vascular endothelium in modulating these responses (see Endothelium earlier in this chapter). Some of the better studied agents are discussed briefly later.
The peptide hormones include vasopressin (arginine vasopressin [AVP] or antidiuretic hormone [ADH]), atrial natriuretic peptide (ANP), vasoactive intestinal peptide, neuropeptide Y, and calcitonin gene–related peptide.44 Of these, AVP and ANP have been the most studied. It has been demonstrated in dogs that AVP, in concentrations 3 to 30 times those found in stressed patients, can cause vasoconstriction sufficient to produce myocardial ischemia.99 In large coronary arteries, the dilator response (via NO) likely exceeded the constrictor response.35 This was due to constriction of the small-resistance vessels. In physiologic concentrations, AVP acts primarily as an ADH with little effect on the coronary circulation. ANP can cause endothelium-dependent coronary dilation but is not known to have significant vascular effects in physiologic concentrations.100
Angiotensin-converting enzyme (ACE) is present on vascular endothelium and converts angiotensin I to angiotensin II (AII), which causes coronary vasoconstriction. AII also facilitates release of norepinephrine from presynaptic adrenergic nerve terminals. ACE inactivates bradykinin, which can attenuate vasoconstriction via NO stimulation. Thus, ACE inhibition can reduce coronary tone by suppressing AII formation and degrading bradykinin, and perhaps also by decreasing norepinephrine release. Despite these theoretical considerations, ACE inhibition has not been shown to be of benefit in human myocardial ischemia other than through control of afterload.101
PGI2 and TxA2 are synthesized from arachidonic acid in a reaction catalyzed by cyclooxygenase. PGI2 is synthesized in the vascular endothelium and, in addition to inhibiting platelet aggregation, induces vasodilation (see Endothelium-Derived Relaxing Factors section earlier in this chapter). TxA2 is mainly synthesized in platelets and causes platelet aggregation and vasoconstriction in the presence of damaged vascular endothelium. In response to TxA2, the intact endothelium releases NO, causing both vasodilation and platelet disaggregation, mechanisms to maintain patency of normal vessels (see Endothelial Inhibition of Platelets section earlier in this chapter). Unlike platelets, the vascular endothelium can synthesize proteins de novo, and thus cyclooxygenase acetylation by aspirin administration has a lesser effect in reducing vascular PGI2 than platelet TxA2
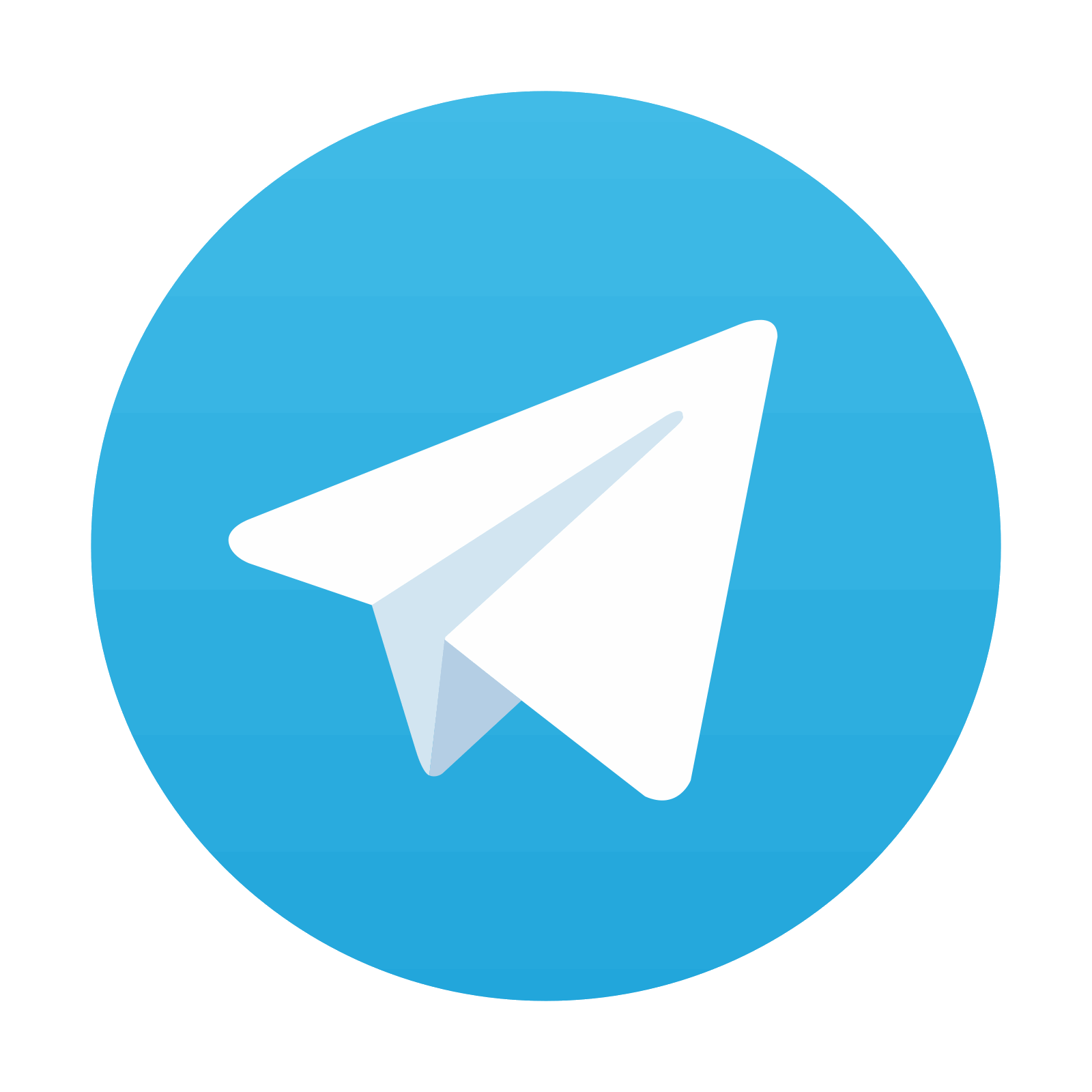
Stay updated, free articles. Join our Telegram channel
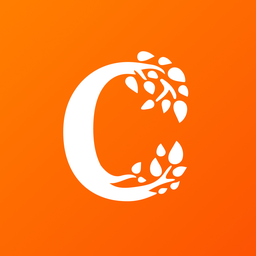
Full access? Get Clinical Tree
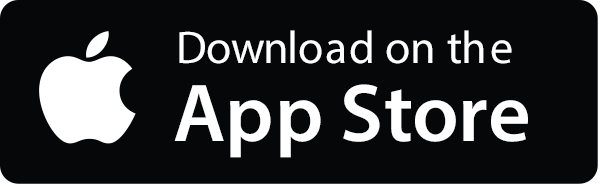
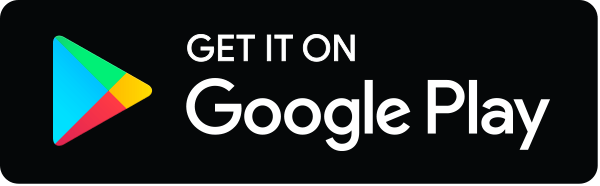