7 Molecular and Genetic Cardiovascular Medicine
The past decades have witnessed what may be termed a revolution in the biomedical sciences, as molecular and genetic methodologies have suddenly jumped onto the clinical scene. Molecular biology originated in the 1950s, its birth most commonly identified with the description of the structure of deoxyribonucleic acid (DNA) by Watson and Crick.1 For many years after, it was practiced almost exclusively in the research laboratory. Much of this research involved the laborious process of cloning: the identification of DNA molecules encoding specific proteins. Although at the time most people in the field realized that these advances would one day be of immense importance to clinical medicine, the exact place they would take was unclear.
The machinery behind the cardiac rhythm: ion channels
The cardiac action potential results from the flow of ions through ion channels, which are the membrane-bound proteins that form the structural machinery behind cardiac electrical excitability. In response to changes in electrical potential across the cell membrane, ion channels open and allow the passive flux of ions into or out of the cell along their electrochemical gradients. This flow of charged ions results in a current, which will alter the cell membrane potential toward the potential at which the electrochemical gradient for the ion is zero and is called the equilibrium potential (E) for the ion. Depolarization of the cell could, in principle, result from an inward cation current or an outward anion current; for repolarization, the reverse is true. In excitable cells, action potentials are mainly caused by the flow of cation currents. Membrane depolarization results principally from the flow of Na+ down its electrochemical gradient (ENa is around +50mV), whereas repolarization results from the outward flux of K+ down its electrochemical gradient (EK is around −90 mV). Opening and closing of ion channels selective for a single ion result in an individual ionic current. The integrated activity of many different ionic currents, each activated over precisely regulated potential ranges and at different times in the cardiac cycle, results in the cardiac action potential. Ion channels are usually highly (but not uniquely) selective for a single ion (e.g., K+ channels, Na+ channels). Channels may rectify, that is, pass current in one direction across the membrane more easily than the other. Electrical and chemical stimuli, which lead to opening and closing of the channel, cause a conformational change in the channel molecule (gating). The rate of change of channel conformation (gating kinetics) may be rapid, in which case the channel will open (activate) almost immediately (e.g., Na+ channels), or relatively slowly, which will result in a delay in channel activation (e.g., delayed rectifier K+ channels). After activation, ion channels may stay open until closed by another stimulus (e.g., repolarization of the membrane) or may close (inactivate) in the face of a continued stimulus. Inactivated channels will usually not reopen on repeat stimulation until they have recovered from inactivation (Box 7-1).

Patch Clamping
The development of the voltage clamp technique in the early 1950s and its application to multicellular preparations of cardiac muscle allowed identification of the major ionic currents that underlie the cardiac action potential. The whole-cell currents recorded by the technique were smooth waveforms derived from summation of the activity of thousands of ion channels, and many different patterns of events at the single-channel level, arising from more than one molecular specie, can summate to produce identical whole-cell current waveforms. Patch clamping allows resolution of events at the single-channel level. In this technique, small patches of cell membrane (< 1 μm2) are isolated electrically and physically in the tip of a glass micropipette.2 Single-channel events can then be resolved because there are only a few ion channel molecules present in the patch. Current flowing across the patch typically jumps between well-defined values corresponding to sudden opening or closing of the ion-conducting pore (Figure 7-1A). The whole-cell current will be the sum of the currents through all of the individual channels in the cell membrane; summation of the current flowing through a single channel during repeated stimuli will reproduce the macroscopic whole-cell current (see Figures 7-1A and B). As channel opening and closing in response to a stimulus are a stochastic phenomena, the regulation of ion flow, whether resulting from a change in membrane potential or from interaction with regulator molecules, is usually achieved by increasing the probability that the channel will be open. Thus, in Figure 7-1, which shows records from human cardiac muscle Na+ channels, the channels open (activate) a few milliseconds after depolarization because the probability that the channel will be open increases. Similarly, as the channels spontaneously close (inactivate), the open probability decreases.

Electrical Events Underlying the Cardiac Action Potential
Figure 7-2 shows a diagram of a cardiac action potential with a summary of the ionic currents flowing during each phase. As far as has been determined, the probable molecular identity of the ion channels that underlie these currents is also given. This section examines the biophysical properties of these currents; subsequent sections focus on possible molecular mechanisms underlying the biophysical phenomena.

Figure 7-2 Ionic currents underlying the cardiac action potential.
(From Roden DM, Lazzarra R, Rosen R, et al, for the SADS Foundation Task Force on LQTS: Multiple mechanisms in the long QT syndrome: Current knowledge, gaps and future directions. Circulation 94:1996, 1996.)
The Resting Membrane Potential and the Role of IK1
The resting cardiac membrane potential is maintained close to the equilibrium potential for potassium (EK) by a background, inwardly rectifying, highly selective K+ current (IK1). Under physiologic conditions, the EK is around −90 mV, and displacement of the membrane potential from this value should result in an inward or outward current through IK1, thus returning the membrane potential toward EK. However, although IK1 passes significant inward current at potentials negative to EK, at positive potentials, the channels display inward rectification (i.e., they pass inward current more easily than outward current), which limits outward current flow and reduces the tendency for IK1 to hyperpolarize the membrane. This has obvious significance for an excitable cell; inward rectification3 allows the initiation of action potentials and limits cellular K+ loss during the action potential. IK1 is large in ventricular cells, smaller in atrial cells, and almost absent in nodal tissue.4 This explains why ventricular cells rest near EK and have a high threshold for excitation, atrial cells have a more positive resting potential, and nodal cells have no defined resting potential.
Phase 0: Rapid Upstroke of the Cardiac Action Potential
The rapid upstroke of the cardiac action potential (phase 0) is caused by the flow of a large inward Na+ current (Ina) (Box 7-2).5 INa is activated by depolarization of the sarcolemma to a threshold potential of −65 to −70 mV. INa activation, and hence the action potential, is an all-or-nothing response. Subthreshold depolarizations have only local effects on the membrane. After the threshold for activation of fast Na+ channels is exceeded, Na+ channels open (i.e., INa activates) and Na+ ions enter the cell down their electrochemical gradient. This results in displacement of the membrane potential toward the equilibrium potential for Na+ ions, around +50 mV. INa activation is transient, lasting at most 1 to 2 msec because, simultaneous with activation, a second, slightly slower conformational change in the channel molecule occurs (inactivation), which closes the ion pore in the face of continued membrane depolarization (see Figures 7-1A and B). The channel cannot open again until it has recovered from inactivation (i.e., regained its resting conformation), a process that requires repolarization to the resting potential for a defined period. Thus, the channels cycle through three states: resting (and available for activation), open, and inactivated. Although the channel is inactivated, it is absolutely refractory to repeated stimulation. Stimuli that occur during recovery from inactivation will result in opening of fewer Na+ channels (because not all have recovered), and the resulting action potential will have a reduced maximal rate of depolarization and reduced conduction velocity. Na+ channels do not need to open to become inactivated. If the resting membrane potential depolarizes for a time, inactivation will occur in some channels and subsequent stimulation will result in an action potential of reduced amplitude and conduction velocity.
Phase 1: Early Rapid Repolarization
The early rapid repolarization phase of the action potential, which follows immediately after phase 0, results both from rapid inactivation of the majority of the Na+ current and from activation of a transient outward current (ITO), carried mainly by K+ ions. On depolarization of the membrane, ITO opens rapidly, over about 20 msec, before spontaneously inactivating. ITO comprises two separate currents: the rapidly inactivating ITO1, which is activated by depolarization and blocked by 4-aminopyridine, and the slowly inactivating ITO2, which is activated by elevated intracellular Ca++ (possibly explaining the observation that action potential duration tends to decrease with rapid heart rates and hypercalcemia)6,7 (Figure 7-3).
In addition to its effect on phase 1, ITO, in combination with the delayed rectifier potassium currents (IKr and IKs) and IK1, also contributes to membrane repolarization. Arrhythmogenic prolongation of the action potential in myocardial cells recovered from patients with myocardial hypertrophy,8 congestive cardiomyopathy,6 and from the border zone of myocardial infarction in animals9 appears to result from depression of ITO.
Phases 2 and 3: Plateau Phase and Final Rapid Repolarization
The action potential plateau and final rapid repolarization are mediated by a balance between the slow inward current and outward, predominantly K+ current. During the plateau phase, membrane conductance to all ions falls and very little current flows. Potassium conductance is low because of inward rectification of IK1 (i.e., inward current passes more easily than outward current), so little outward current flows despite the large outward electrochemical gradient for K+ ions and the delayed onset of the outwardly rectifying K+ currents (IKs, IKr, and IKur). The resulting small outward current is balanced by inward current, predominantly through L-type Ca++ channels (ICa-L), but also via a slowly inactivating population of Na+ channels, and a small inward flux of chloride (Cl–) ions, possibly carried by the cardiac variant of the ATP-dependent channel (abnormalities of which underlie cystic fibrosis).10,11 Phase 3, regenerative rapid repolarization, results from time-dependent inactivation of L-type Ca++ current and increasing outward current through delayed rectifier K+ channels. The net membrane current becomes outward and the cell repolarizes.
Slow Inward Ca++ Current
The slow inward current (ICa-L) is activated by depolarization of the cell to potentials less negative than −40 to −50 mV. In ventricular and atrial myocytes and in Purkinje fibers, ICa-L is activated by the regenerative depolarization caused by INa during phase 0 of the action potential. ICa-L does not contribute significantly to phase 0 because, in comparison with INa, it activates much more slowly (over about 10 msec) and is smaller in amplitude. ICa-L also inactivates slowly and, therefore, contributes the major inward current during the plateau of the action potential. ICa-L flows through L-type (long-lasting) Ca++ channels, which are sensitive to block by dihydropyridines (e.g., nifedipine), and activation of contraction is related to the magnitude of the resulting calcium influx.12 Gating of ICa-L is generally similar to INa in that channel opening and closing are dependent on membrane potential and time. Ca++ channels are also, importantly, dynamically regulated by the autonomic nervous system.13 β agonists activate ICa-L (and hence increase myocardial contractility) indirectly by activating adenylyl cyclase via a guanosine triphosphate (GTP)–binding protein, Gs (Figure 7-4). The resulting increase in intracellular cyclic adenosine monophosphate (cAMP) activates protein kinase A (PKA), which phosphorylates the Ca++ channel. Phosphorylated channels open in response to membrane depolarization; nonphosphorylated channels do not, so the effect of β-adrenergic stimulation is to increase the number of functional channels. The electrophysiologic effect of this is illustrated in Figure 7-5, which shows enhancement of the slow inward current by increase of adenosine monophosphate (AMP) level in single-channel Ca++ channels and intact cells. β-Adrenergic effects on ICa-L are antagonized by acetylcholine, which, in myocardial cells, activates M2 muscarinic receptors and inhibits adenylyl cyclase through activation of the GTP-binding protein Gi.

Figure 7-5 Effects of elevated cyclic adenosine monophosphate (cAMP) on cardiac calcium channels.
(A, B, Modified from Rampe D, Lacerda AE, Dage RC, Brown AM: Parathyroid hormone: An endogenous modulator of cardiac calcium channels. Am J Physiol 261[6 Pt 2]:H1945, 1991.)
Delayed Rectifier K+ Currents
Delayed rectifier K+ channels are present in all cardiac myocytes. They open slowly (over 200 to 300 msec) after depolarization of the membrane to the plateau level (−10 mV and greater), producing a K+-selective outward current, IK. IK does not inactivate on prolonged depolarization (unlike INa and ICa-L), and the channels close on repolarization of the membrane. Unlike IK1, IK displays outward rectification; that is, it passes outward current more easily than inward current. This is the expected behavior for a K+-selective channel because both the concentration and electrical gradients for potassium are outward. Thus, for any depolarizing displacement of membrane potential from EK, the driving force will be larger in an outward direction. Similar to ICa-L, IK is under autonomic control (see Figure 7-4). β-Adrenergic stimulation enhances IK by a mechanism similar to that of the enhancement of ICa-L, thus ensuring repolarization of the cell in the face of increased inward Ca++ current.14
Three components of IK, carried by different channel molecules, can be distinguished. A rapidly activating component, IKr, is blocked by the compound E4031 (a Class III antiarrhythmic agent), which leaves a slower activating component, IKs, unaffected.15 This is illustrated in Figure 7-6, which also emphasizes the importance of IK in the regulation of repolarization, and hence of action potential duration. A third component, the ultra-rapidly activated delayed rectifier, IKur, can be distinguished in atrial (but not ventricular) myocytes.16 This additional repolarizing current explains, in part, the enhancement of repolarization in atrial myocardium when compared with ventricle and Purkinje fibers.
Repolarization in Different Cardiac Tissue Types
Phase 3 repolarization in atrium and pacemaker tissues, but not in ventricular myocardium, is also enhanced by the presence of a large outward repolarizing K+ current (IK[ACh]).17,18 This potential independent current is activated indirectly by stimulation of muscarinic (M2-type) receptors by acetylcholine or purinergic (A-type) receptors by adenosine.19 This channel is potential independent and is activated via binding of an activated, membrane-bound GTP-binding protein (Gi), as discussed later.20
There is variability in action potential duration between cells in normal ventricle.21 A gradient in action potential duration exists across the myocardium (from epicardium to endocardium), and specialized midmyocardial cells (M cells) have been identified, which exhibit prolongation of action potential duration at slow stimulation rates, possibly as a result of a decrease in IKs.
Phase 4: Diastolic Depolarization and If
Phase 4 diastolic depolarization, or normal automaticity, is a normal feature of cardiac cells in the sinus and atrioventricular (AV) nodes, but subsidiary pacemaker activity is also observed in the His-Purkinje system and in some specialized atrial and ventricular myocardial cells (see Chapter 4). Pacemaker discharge from the sinus node normally predominates because the rate of diastolic depolarization in the sinoatrial (SA) node is faster than in other pacemaker tissues. Pacemaker activity results from a slow net gain of positive charge, which depolarizes the cell from its maximal diastolic potential to threshold.
Pacemaker cells in the sinus node are relatively depolarized, with a maximal diastolic potential of −60 to −70 mV and a threshold potential of −40 mV. Rapid regenerative depolarization (phase 0) is dependent on opening of T-type and then L-type Ca++ channels. Repolarization is dependent on activation of delayed rectifier K+ channels, and the maximum diastolic potential is around −80 mV. Pacemaker channels are activated by hyperpolarization to this potential and produce a slow inward Na+ current, If. This flows against slowly inactivating delayed rectifier K+ currents and results in diastolic depolarization.22 Because the current is nonselective among cations, its reversal potential lies between EK and ENa, at around −10 mV, and activation of If will tend to depolarize the cell toward this value. Similar to ICa-L, If is under autonomic control (see Figure 7-4) through GTP-dependent binding proteins Gs and Gi, which regulate cAMP production by adenylyl cyclase.23,24 β-Adrenergic stimulation shifts the voltage dependence of activation of If to more depolarized potentials, so for any hyperpolarizing stimulus, more If will be activated and diastolic depolarization will be enhanced. Acetylcholine has the opposite effect (Figure 7-7).

Molecular Biology of Ion Channels
The preceding sections have focused on the electrical events that underlie cardiac electrical excitability, and on the identification of cardiac ionic currents on the basis of their biophysical properties. Here the molecular structures behind these electrical phenomena are reviewed. The first step in understanding the molecular physiology of cardiac electrical excitability is to identify the ion channel proteins responsible for the ionic currents. Figure 7-2 gives the current classification of the ion channel responsible for each of the cardiac ionic currents. There are firm molecular candidates for voltage-gated Na+ and L-type Ca++ channels. Similarly, channel molecules with properties similar to delayed rectifier K+ channels, the 4-aminopyridine–sensitive component of ITO, the inward rectifier IK1, the ligand-gated K+ channel IK(ACh), and the pacemaker current If have been cloned. Figure 7-8 shows diagrams of the predicted membrane topology of some of these channels. Voltage-gated Na+, Ca++, and K+ channels exist as conglomerates of molecules, consisting of a large α subunit and several accessory subunits (labeled β, δ, and γ in Figure 7-8). The α subunit alone is usually sufficient to induce channel activity in biologic membranes, but its activity is modulated by the presence of the accessory subunits. The diagrams in Figure 7-8 were deduced from hydrophobicity analysis of the primary structure of the major channel polypeptides. Regions of the polypeptides predicted to span the membrane are those that contain a high concentration of hydrophobic amino acids, whereas peptides linking these transmembrane sections are hydrophilic. Similarities among the various channels strongly suggest a common evolutionary ancestry. Na+ and Ca++ channel α subunits (see Figures 7-8A and B) are strikingly similar, each consisting of four homologous transmembrane domains (labeled I to IV), linked by cytoplasmic peptides. Each homologous domain contains six linked membrane-spanning segments (labeled S1 to S6). These large polypeptides, containing more than 2000 amino acids, form a tetrameric structure and generate Na+ or Ca++ channel activity in biologic membranes. Voltage-gated K+ channel α subunits, in contrast, are much smaller (see Figure 7-8C) and consist of a single transmembrane domain with six membrane-spanning segments, an arrangement similar to one of the individual domains of Na+ and Ca++ channels. Four molecules are noncovalently linked in the membrane to produce a tetrameric structure, similar to a Na+ or Ca++ channel, to produce K+ channel activity. The structure of the inwardly rectifying K+ channel molecules, IK1 and IK(ACh), is dissimilar to other K+ channels (see Figure 7-8C). The molecules are much less complex, having only two membrane-spanning segments, although these segments share considerable homology with the S5 and S6 segments of the classic voltage-gated K+ channel.

Figure 7-8 Diagrams of ion channel molecular structure.
A, Na+ channel. B, Ca++ channel. C, K+ channels. ATP, adenosine triphosphate.
Molecular Mechanisms
The Voltage Sensor
Channel proteins respond to changes in electrical potential across the cell membrane by conformational changes (gating), which result from electrostatic interactions between charged portions of the molecule and the membrane electric field (Box 7-3). Gating of the channel is associated with a measurable flow of electrical charge through the membrane lipid bilayer (called gating current), as a zone of the molecule rich in electrical charge moves within the membrane.25 This charge movement is linked to opening of the channel pore. The voltage sensor of voltage-dependent ion channels resides in the mobile S4 membrane-spanning segments, α-helical structures unusually rich in positively charged amino acids.26 At rest, each of the positive charges in the S4 segment is balanced by fixed negative charges in other segments of the molecule. The resting membrane potential (negative inside) forces the (mobile) positive charges inward and the fixed negative charges outward. This dynamic equilibrium holds the channel pore closed. On depolarization, the force pulling the positive charge inward is relieved; positive charges (the S4 segments) are repelled outward and assume new partners with the fixed negative membrane charges. This charge movement comprises the gating current. If the depolarizing stimulus is short, repolarization of the membrane is followed by a gating current of equal and opposite magnitude as the S4 segment relaxes to its original position. If the depolarizing stimulus is prolonged, however, the movement of the S4 segments induces a conformational change in the channel molecule, which prohibits easy return to baseline. This conformational change in the channel molecule is manifested as activation (or channel opening), and this is closely coupled to channel closing (or inactivation; see later) in channels that inactivate. Thus, small changes in the membrane electric field cause conformational changes in the channel molecule, which result in opening (and closing) of the channel pore. An S4 segment rich in positive charge is a remarkably consistent feature of voltage-gated ion channels from a variety of different species and with a variety of ion selectivities. The dependence of channel activation on membrane potential is proportional to the density of positive charge in the S4 segment.
Ion Channel Pore and Selectivity Filter
The presence of four homologous domains in voltage-gated Na+ and Ca++ channels suggests that basic ion channel architecture consists of a transmembrane pore surrounded by the four homologous domains arranged symmetrically (see Figure 7-8). The membrane-spanning segments each form an α helix so that the walls of the pore will be derived from α-helical segments from each of the four domains. A pore formed from four such α helices would have limiting dimensions of 3 by 5 Angstrom units, similar to the size inferred for the Na+ channel pore by measurement of the permeability of cations of different sizes.27,28
The selectivity filter is formed by the S5 and S6 membrane-spanning segments of each domain together with their peptide linker.29 As emphasized in Figure 7-8, unlike the hydrophilic extracellular linkers between other membrane-spanning segments, the S5/S6 linker is sufficiently hydrophobic to place it (at least partially) within the membrane lipid bilayer. The channel pore is lined both by the S5/S6 linker and the S5 and S6 membrane-spanning segments. Point mutations in the S5/S6 linker have dramatic effects on channel ion selectivity and reduce channel conductance to its primary ion. Extensive site-directed mutagenesis experiments of the S5/S6 linkers from a variety of channels suggest that they form a funnel that allows the passage of a specific ion into the pore. In Na+ channels, selectivity is imposed by two rings of negatively charged amino acids at the outer mouth of the funnel, which collect Na+ ions for transmission into the cell.27
Channel Inactivation
Inactivation gating is the process by which ion channels close in the face of continuing depolarization. Inactivation is characteristic of voltage-gated Na+ and Ca++ channels, as well as the K+ channels underlying ITO. Inactivation begins after activation gating, as a second, slower conformational change in the molecule that halts the ion flux through the channel. Inactivation gating is thus closely coupled to activation gating, and ionic current flows only while both the activation and inactivation gates are open simultaneously. In Na+ channels, the inactivation gate is formed by the intracellular peptide linker between homologous domains III and IV (Figure 7-9A).30 This peptide is postulated to act as a hinged lid, which moves upward to plug the ion pore (and thus halt current flow) shortly after membrane depolarization.26 For the channel to recover from inactivation (i.e., to be ready to open in response to a new depolarizing stimulus), the III/IV linker peptide must resume its resting position, a process that requires hyperpolarization of the membrane to the resting potential for a finite period. Site-directed mutagenesis of the III/IV linker peptide has revealed a trio of hydrophobic amino acid residues (isoleucine, I; phenylalanine, F; and methionine, M), near to the domain III end of the peptide, which are crucial for normal channel inactivation. Replacement of just one of these residues (the phenylalanine) almost completely removes inactivation. These residues are postulated to latch on to a receptor in the channel pore to close the channel. The molecular basis of inactivation in K+ channels is rather different from Na+ channels. Because the four domains of K+ channels are formed by noncovalently linked molecules, there are no interdomain linkers to plug the channel pore. In K+ channels, a picture of an N-terminal ball-and-chain mechanism has emerged (see Figure 7-9B).31 The terminal 20 or so amino acids are very hydrophobic and are postulated to swing up and attach to the open pore. The next few amino acids contain a number of positively charged residues that draw the whole N-terminal end up to the membrane. These two domains act as a ball. The remaining amino acids, up to the beginning of the transmembrane S1 segment, act as a chain. If the chain is made longer, inactivation is slower, and vice versa.

Clinical Correlates
Ion Channels and Antiarrhythmic Drugs
Drug therapy of cardiac arrhythmias would ideally be targeted at an individual ionic current, tailoring the cardiac action potential in such a way that abnormal excitability was reduced but normal rhythmicity was unaffected. This remains an as-yet unrealized goal. The prototype antiarrhythmic agents (e.g., disopyramide and quinidine) have diverse effects on cardiac excitability and, similar to agents introduced more recently, frequently exhibit significant proarrhythmic activity with potentially fatal consequences. In the Cardiac Arrhythmia Suppression Trial (CAST), mortality among asymptomatic postmyocardial infarction patients was approximately doubled by treatment with the potent Na+-channel–blocking agents encainide and flecainide—an effect likely attributable to slowing of conduction velocity with a consequent increase in fatal reentrant arrhythmias.32 The results of the CAST prompted efforts to approach antiarrhythmic drug therapy by prolonging action potential duration (e.g., dofetilide)—a strategy with some support from animal studies, but one that also may cause proarrhythmia through induction of polymorphic ventricular tachycardia (the acquired long QT syndrome [LQTS]).33 Drugs that prolong action potential duration all block IKr, and it is not clear that this therapeutic goal will result in arrhythmia control without induction of clinically significant proarrhythmia. The only drugs currently available that definitely prolong life by reducing fatal arrhythmias are β-blockers (e.g., ISIS-1, 1997), and these agents have no channel-blocking effects.
Ion Channels in Disease
Long QT Syndromes
This rare group of ion-channel abnormalities causes abnormal prolongation of the cardiac action potential, resulting in early afterdepolarizations (i.e., oscillations in the action potential during the plateau phase) and death from polymorphic ventricular tachycardia (torsades de pointes type). To date, six LQTS subtypes have been identified on the basis of the affected gene. They are numbered sequentially according to the date of discovery (Table 7-1), and all the loci so far identified encode ion channels except for LQT4. LQTS occurs because of disruption of cardiac repolarization, and in principle this can occur either from enhancement of inward depolarizing current or reduction of outward current. LQT3 is a gain-of-function mutation of the cardiac Na+ channel that results in failure of channel inactivation, most commonly from a deletion of three amino acids from the inactivation gate. LQT1 and LQT2 result from mutations of the delayed rectifier K channels that underlie IKs and IKr, and LQT5 and LQT6 result in reductions of IKr and IKs through mutations in channel accessory subunits. Loss of repolarizing current results in prolongation of the QT interval, and this leads to the syndrome. LQT4 is unique in that it results from a mutation in ankyrin B, an adapter protein that binds to the Na+ pump and Na+/Ca++ exchanger. The resulting effects on cellular Ca++ homeostasis cause ventricular arrhythmias.34 Identification of the molecular substrate for LQTS will allow detection of the disease in asymptomatic carriers and may in the future allow targeted gene therapy; these issues are discussed in more detail later in the Genetic Cardiovascular Medicine section.
Brugada Syndrome
The Brugada syndrome also is a group of ion-channel abnormalities that affect cardiac repolarization and result in cardiac sudden death. It is characterized by incomplete right bundle-branch block and persistent ST-segment elevation in the anterior precordial leads of the electrocardiogram (ECG).35 Cases for which the genotype is available appear to result from channel mutations that reduce depolarizing Na+ current. This results in loss of the action potential dome, an effect that is most marked in the right ventricular epicardium where the transient outward current ITO1 is strongly expressed (hence the ST-segment elevation in the anterior chest ECG leads). Early repolarization of the epicardial action potential results in a transmural repolarization gradient, and this can lead to reentry and sudden cardiac death.36 In more than two thirds of patients with Brugada syndrome, the genetic locus is unknown, and much work remains to be done to elucidate the mechanism of this condition.
Controlling cardiac functioning: receptors

Receptors
Receptors are grouped in several broad classes, the protein tyrosine kinase receptors and the G-protein–coupled receptors (GPCRs) being the most important ones. The protein tyrosine kinase receptors are large molecular complexes that incorporate phosphorylating enzyme activity in the intracellular segment. Ligand binding induces activation of this enzyme activity. Because phosphorylation is one of the major mechanisms of cellular regulation (see, for example, the phosphorylation of the Ca++ channel described earlier), such receptors can have a variety of cellular effects (Box 7-4). GPCRs are much smaller than protein tyrosine kinase receptors. Ligand binding results in activation of an associated protein (G protein)
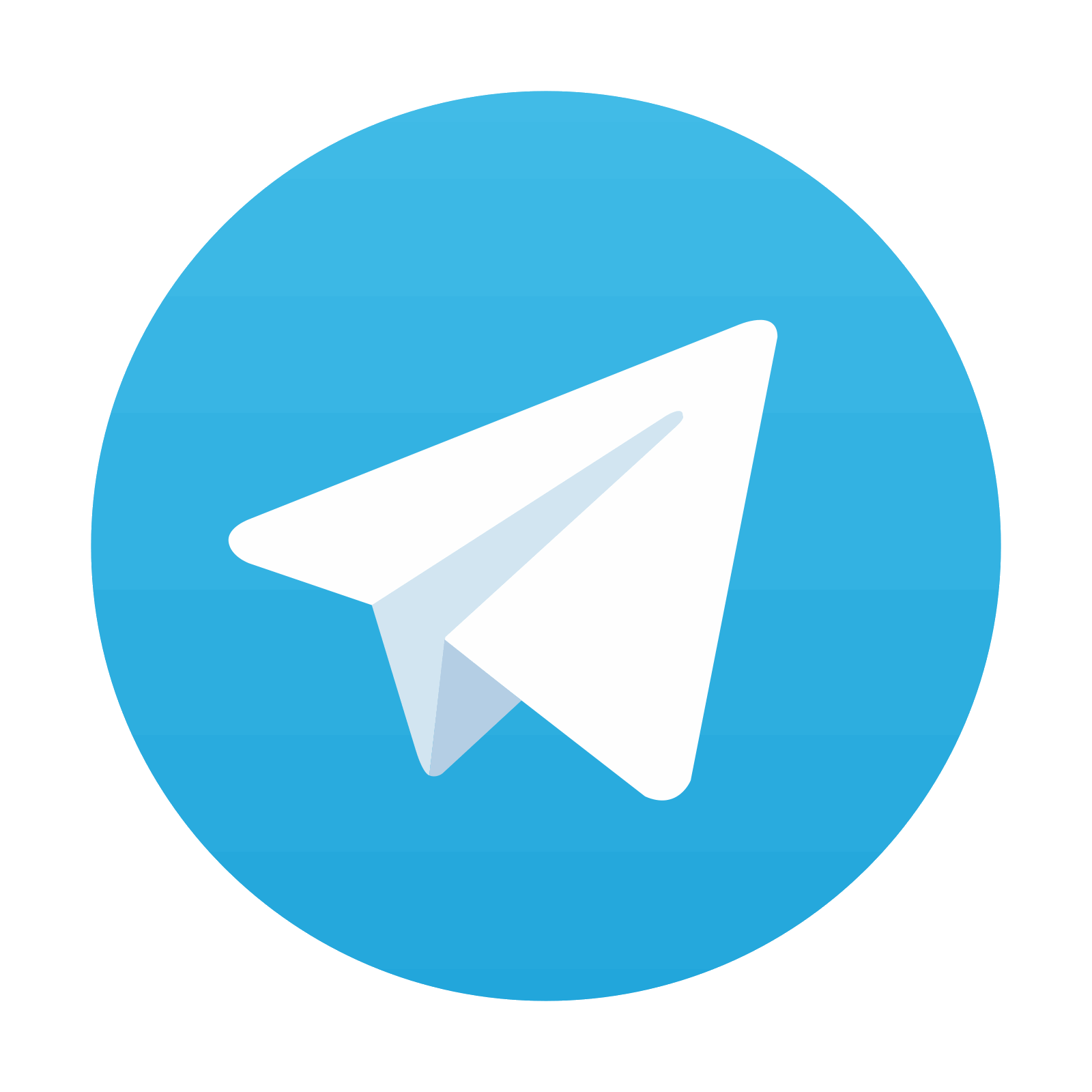
Stay updated, free articles. Join our Telegram channel
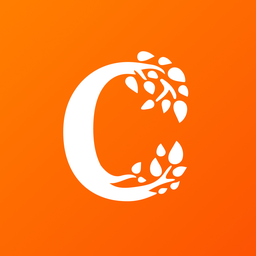
Full access? Get Clinical Tree
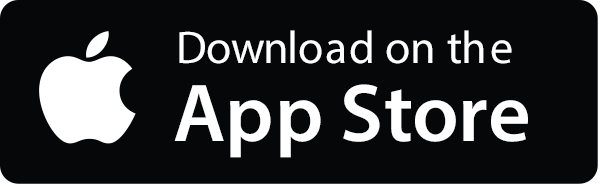
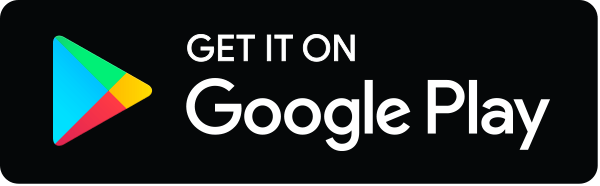