Key Points
- ▪
Mechanisms of consciousness and memory, and their interruption by general anesthetics, are important scientific problems that have clinical relevance for the practice of anesthesiology.
- ▪
Consciousness is characterized by both wakefulness (i.e., the brain being aroused) and awareness (i.e., subjective experience).
- ▪
Anesthetics act at structures in the brainstem, hypothalamus, and basal forebrain that regulate sleep-wake states, which may account for loss of wakefulness.
- ▪
Anesthetics disrupt connectivity and communication across cortical and thalamocortical networks, which may account for loss of awareness.
- ▪
Memory can be subdivided into explicit (conscious) and implicit (unconscious) recall; an example of explicit episodic recall is remembering a surgical event.
- ▪
Suppression of explicit episodic recall is one of the most potent effects of most general anesthetics.
- ▪
Effects on the hippocampus, amygdala, and prefrontal cortex—as well as the connectivity of these structures—may account for anesthetic-induced amnesia, even before loss of consciousness.
Introduction
Scientific and Clinical Importance
Consciousness and memory are among the most fascinating and complex subjects in all of science. The richness of human consciousness and memory—and the ability to express this richness in language—is a defining characteristic of homo sapiens . Consciousness and memory also have clinical relevance for the anesthesiologist; together, the experience and explicit episodic recall of surgical events is known as the problem of “intraoperative awareness.” When formally assessed, this complication occurs in approximately 1 to 2 cases per 1000 and is associated with a high incidence of posttraumatic stress disorder (PTSD). The incidence of intraoperative consciousness without recall is substantially higher. To advance the field of perioperative brain monitoring, a detailed understanding of the neurobiology of consciousness, memory, and anesthesia is required.
Consciousness
Definitions
The field of consciousness studies has been plagued by the indiscriminate use of the term “consciousness.” When we refer to consciousness, we mean subjective experience . In simple terms, it is what we lose when we have dreamless sleep and what we regain again in the morning upon awakening. There are, however, several important definitions and distinctions that should be considered.
- 1.
Awareness : Cognitive neuroscientists and philosophers use the term “awareness” to mean only subjective experience. In clinical anesthesiology, we (inaccurately) use the term “awareness” to include both consciousness and explicit episodic memory (the taxonomy of memory will be discussed in the next major section of the chapter).
- 2.
Connected versus disconnected consciousness : Connected consciousness is the experience of environmental stimuli (such as surgery), whereas disconnected consciousness is an endogenous experience (such as a dream state).
- 3.
Consciousness versus responsiveness : An individual may fully experience a stimulus (such as the command “Open your eyes!”) but not be able to respond (as when a patient is paralyzed but conscious during surgery).
There have been a number of theories proposed to explain the mechanisms of consciousness and general anesthesia. Advances in neuroscience, however, have enabled us to move beyond speculative frameworks and focus on a systems-based approach to both subjects. The remainder of this section on consciousness adopts such an approach by discussing (1) brainstem and hypothalamic nuclei regulating the sleep-wake cycle (and therefore arousal states) ( Figs. 9.1 and 9.2 ); (2) the role of the thalamus in consciousness and anesthesia; (3) cortical-subcortical connectivity, with a focus on the thalamocortical system, which is thought to mediate the experiential component of consciousness; and (4) corticocortical communication.


Subcortical Nuclei Regulating Arousal
It was hypothesized in the mid-1990s that anesthetics suppress consciousness by actions at the subcortical nuclei that evolved to control sleep-wake cycles. The past decades have supported the hypothesis that anesthetics interact with a number of these sleep-wake centers, although precise interactions and contributions to the state of general anesthesia have yet to be elucidated. The following is a description of select subcortical nuclei in the brainstem and hypothalamus that mediate sleep-wake cycles and, potentially, some traits of anesthesia.
Brainstem
Locus ceruleus
Norepinephrine is synthesized in the locus ceruleus (LC), which is located in the pons and projects widely throughout the cortex. Like other monoaminergic neuronal populations, LC activity is highest during waking consciousness, decreased during nonrapid eye movement (NREM) sleep, and at its nadir during rapid eye movement (REM) sleep. Thus LC is associated with cortical arousal only during wakefulness and not with the cortical activation during REM sleep. LC neurons are hyperpolarized by halothane. The role of norepinephrine (generated by LC) in anesthesia is further supported by studies demonstrating that barbiturate anesthesia time is increased by antagonizing norepinephrine and reduced by agonizing it. Norepinephrine transmission in the basal forebrain may be of particular relevance to anesthetic depth. It has been found that LC noradrenergic neurons modulate the state of isoflurane anesthesia as well as emergence therefrom. Of note, administration of ketamine is associated with an increase of activity in the LC and appears to contribute to its anesthetic effects.
LC and the role of norepinephrine in hypnosis are of particular interest due to the role of the α-2 agonist dexmedetomidine in clinical care. Microinjection of dexmedetomidine in the LC results in reduced levels of consciousness that can be prevented by coadministration of the α-2 antagonist atipamezole. After exposure to dexmedetomidine, brain changes somewhat mimic NREM sleep in that the LC and tuberomammillary nucleus (TMN) are deactivated, whereas the ventrolateral preoptic nucleus (VLPO) is activated. Data in dopamine-β-hydroxylase knockout mice (which lack the ability to synthesize norepinephrine) demonstrate a hypersensitivity to dexmedetomidine, suggesting alternative mechanisms of action. However, selective knockdown of α-2A adrenergic receptors from LC prevent dexmedetomidine-induced loss of righting reflex, a marker of general anesthesia in rodents.
Laterodorsal/pedunculopontine tegmentum
Along with the basal forebrain, the laterodorsal tegmentum (LDT) and pedunculopontine tegmentum (PPT) in the pons are the brain’s source of acetylcholine. There are direct projections to the thalamus from LDT/PPT with a known role in the generation of slow oscillations and sleep spindles, which together represent a neurophysiologic sign that information transfer to the cortex is likely blocked. As with the noradrenergic LC, activity of the LDT/PPT is high during waking consciousness and decreases during NREM sleep. However, in contrast to the LC and other monoaminergic neurons, the cholinergic LDT/PPT is also active during REM sleep, during which the cortex is aroused. Furthermore, activation of cholinergic neurons in LDT or PPT induces REM sleep. Thus, both states of cortical activation across the sleep-wake cycle are associated with high cholinergic tone. General anesthetics modulate cholinergic projections from the LDT/PPT. Sleep spindles occur during halothane anesthesia and are associated with decreased cholinergic transmission to the medial pontine reticular formation (PRF). There is evidence that synaptic and extrasynaptic γ-aminobutyric acid (GABA) receptors play a role in modulating LDT neurons, which could provide a direct link to molecular mechanisms of numerous general anesthetics. However, there has been relatively little study of the role of LDT/PPT in anesthetic mechanisms, with a greater focus on cholinergic neurons in the basal forebrain.
Pontine reticular formation
The PRF is part of the reticular activating system, which plays an important role in cortical arousal. Although GABA is the primary inhibitory neurotransmitter in the brain, the actions of GABA in the PRF are associated with cortical arousal. For example, there is increased time spent in the waking state when the GABA A receptor agonist muscimol is microinjected in the PRF. When the GABA A antagonist bicuculline is microinjected, wakefulness is suppressed, but REM sleep (another state of cortical arousal) is triggered. Vanini and colleagues found that decreased levels of GABA in the PRF correlated with isoflurane-induced unconsciousness, muscular hypotonia, and decreased respiratory rate. Since the effects of anesthetics are normally associated with a potentiation of GABA activity, these findings highlight that a specific neuroanatomic and neurochemical milieu can play a unique and unexpected role in the mechanisms of consciousness and anesthesia. In addition, Vanini and colleagues found that GABA-ergic transmission in the rat PRF modulates the loss of consciousness induced by isoflurane but does not appear to affect emergence, providing evidence for asymmetry between the two processes.
The mesopontine tegmental anesthesia area is located in the PRF. When pentobarbital is microinjected in this area, a reversible state with anesthetic traits is induced. More recently, this phenomenon has been defined with greater spatial resolution, with the identification of around 1900 neurons in this area that can induce general anesthesia.
Ventral tegmental area
Dopaminergic neurons of the ventral tegmental area (VTA) in the midbrain have not classically been considered key mediators of the sleep-wake cycle because of relatively less evidence of state-dependent changes compared with neurons in other brainstem nuclei. This view has been challenged in sleep neurobiology. A dopaminergic pathway regulating sleep-wake states has been identified in Drosophila and dopaminergic neurons of the VTA have more recently been found to play a role in mammalian sleep. There has been renewed interest in the ability of dopaminergic activity to reverse or accelerate recovery from general anesthesia. Studies of the dopamine agonist methylphenidate have revealed an ability to reverse the sedative effects of both isoflurane and propofol. VTA appears to be the source of the dopaminergic transmission mediating arousal during exposure to anesthesia, as evidenced by the fact that electrical stimulation of the VTA or selective stimulation of VTA dopaminergic neurons can reverse anesthetic-induced unconsciousness.
Hypothalamus
Ventrolateral preoptic nucleus
The anterior hypothalamus has long been hypothesized to play a role in sleep-wake regulation. VLPO is a structure in this region that transmits GABA and galanin. Neurons in VLPO are maximally active during NREM and REM sleep; the median preoptic nucleus (MnPO) is also active during sleep. Of note, the activity profile of GABA-ergic neurons in VLPO correlates with sleep amount, whereas the activity of GABA-ergic neurons in MnPO correlates with homeostatic sleep pressure or propensity. Importantly, activity of the VLPO during sleep is correlated with inhibition of other arousal centers in the brainstem and hypothalamus. Given its potentially central role as a mediator of sleep, VLPO became an attractive candidate as a mediator of anesthetic-induced unconsciousness. Nelson and colleagues demonstrated activation of VLPO after systemic administration of propofol or pentothal ; recent studies have examined the mechanistic significance of these findings. Eikermann and colleagues conducted studies of rats with chronic lesions of VLPO, finding that ablation of VLPO resulted in sleep deprivation (as expected) but conferred increased sensitivity to the effects of isoflurane. This finding would argue against a critical role of VLPO in the mechanism of anesthesia. However, Moore and colleagues demonstrated that acute lesions of VLPO conferred resistance to the effects of isoflurane, an effect that appeared to be mediated specifically through the sleep-active neurons in VLPO. These neurons are actually depolarized (i.e., activated) by isoflurane. Taken together, these data suggest that VLPO plays a role in anesthetic-induced unconsciousness (as evidenced by acute lesion data), but that the effects of sleep deprivation associated with chronic VLPO lesions could overwhelm this role. Curiously, direct administration of dexmedetomidine, an α-2-adrenergic agonist, to the VLPO can destabilize the state of isoflurane anesthesia.
Orexinergic neurons
Orexinergic neurons are found in the lateral hypothalamus and provide an important arousal stimulus for the cortex. There are two types of orexin (A and B), which are also referred to as hypocretins. Orexinergic neurons innervate other arousal centers in the brainstem and basal forebrain, and fire maximally in the waking state, are suppressed during NREM sleep, and show occasional bursts during phasic REM sleep. Dysfunction of the orexinergic system is associated with narcolepsy in both human and animal models. The often dramatic delay of anesthetic emergence in narcoleptic patients has motivated the study of orexin in anesthetic mechanism. Orexins attenuate the effects of isoflurane, propofol, ketamine, and barbiturates, using various measures. Local infusion of orexin in the basal forebrain is associated with electroencephalographic (EEG) arousal and decreased emergence time in animals anesthetized with sevoflurane and isoflurane. Microinjection of propofol in the perifornical region of the hypothalamus (the locus of orexinergic neurons) is associated with a decrease in cortical acetylcholine, an important mediator of arousal. Importantly, both genetic and pharmacologic studies have demonstrated that orexins play an important role in emergence from sevoflurane and isoflurane anesthesia, but not induction. This seminal study suggested that there is a distinct neurobiology of induction and emergence, and formed the basis for a theory of “neural inertia” across state transitions. Of note, halothane did not show an effect on orexinergic neurons and emergence time was not altered in orexin knockout mice. These findings have been confirmed for propofol anesthesia: propofol reduces activity in orexinergic neurons in rats and infusion of orexin in the basal forebrain affects emergence time, but not induction time. Orexins might facilitate emergence from anesthesia through actions in the basal forebrain.
Tuberomammillary nucleus
The TMN is located in the caudal hypothalamus and is the brain’s source of histamine, an arousal-promoting transmitter. TMN activity and histamine levels are highest during wakefulness and lowest during sleep; the TMN is thought to have a relationship of reciprocal inhibition with the sleep-promoting GABA-ergic neurons of the VLPO. Histamine release in the anterior hypothalamus is depressed during sleep and halothane anesthesia. Systemic administration of propofol, pentothal, and the GABA agonist muscimol all result in decreased activity in the TMN. Microinjection of histamine in the nucleus basalis magnocellularis of the basal forebrain reverses the depressant effects of isoflurane on the EEG, an effect likely mediated by H1 histamine receptors. A recent study in which GABA A receptors were genetically removed demonstrated that histaminergic neurons are resistant to the effects of propofol. However, at the behavioral level, there was no effect of propofol on loss-of-righting reflex, a surrogate for anesthetic-induced unconsciousness. Thus the role of TMN and histaminergic transmission in the mechanism of anesthesia is still unclear.
Role of the Thalamus
The thalamus is composed of more than 50 nuclei and subnuclei that can be grossly classified as relays for sensory input from the periphery or multimodal, integrative regions that receive input from the cortex. Additionally, the thalamus is critical for transmitting arousal signals from the brainstem and for regulating cortical communication. The involvement of the thalamus in arousal, sensory processing, and cortical computation is likely critical for normal consciousness. As such, the thalamus has been of continued interest to those investigating mechanisms of anesthetic-induced unconsciousness.
The thalamus has been proposed as an ON/OFF switch for anesthetic state transitions. This theory was generated based on the consistent metabolic depression of the thalamus by a number of inhaled and intravenous anesthetics (with the exception of ketamine), suggesting that it could serve as an effective OFF switch. The hyperpolarization of the thalamus would shift tonic firing to burst firing that—as with sleep—would prevent afferent sensory stimuli from arousing the cortex. Importantly, however, sensory nuclei in the thalamus (and their connectivity to the cortex) appear to be less involved in anesthetic-induced unconsciousness compared to the higher-order or “nonspecific” nuclei.
Evidence for the thalamus as an ON switch has been derived primarily from animal experiments, in which stimulation of the centromedial thalamus by either nicotine or antibodies blocking voltage-gated potassium channels could reverse the effects of inhaled anesthetics. Although microinjection of large doses of nicotine into the thalamus could precipitate anesthetic emergence, antagonism of nicotinic acetylcholine receptors in the same location did not appear to contribute to anesthetic-induced unconsciousness. Central thalamic activation results in behavioral improvement in humans with traumatic brain injury. A study in humans demonstrated that (spontaneous) activation of the thalamus along with other subcortical structures is correlated with recovery from anesthesia, suggesting the involvement of the thalamus in the primitive or “core” consciousness observed at emergence.
Nonspecific nuclei of the thalamus have been proposed as a computational blackboard for the cortex. Thus if the mechanism of anesthetic-induced unconsciousness was achieved primarily by a suppression of cortical computation, a depressed thalamus should be the result. To address this question, Velly and colleagues conducted a neurophysiologic study using scalp EEG (reflecting cortical signals) and subthalamic nuclei electrodes (argued to reflect thalamic activity). Induction of anesthesia with either propofol or sevoflurane was associated with cortical rather than subcortical changes, suggesting that the depression of the thalamus identified through neuroimaging studies reflected an effect rather than a cause of anesthetic-induced unconsciousness. Other case studies in humans suggest concurrent suppression of thalamus and cortex during induction of propofol. Furthermore, more precise neurophysiologic recordings of thalamus and cortex in animal models suggest that effects of propofol on central thalamic activity precede effects on the cortex; interestingly this was found during the spontaneous induction of sleep as well. Other animal studies have found that attenuation of high-frequency oscillations by propofol is more pronounced in the thalamus than the cortex.
The prior two possibilities (thalamic switch or computational blackboard for the cortex) treat the thalamus as a passive player in general anesthesia. However, more recent data suggest that it might, instead, play an active role. A computational study using human EEG data and modeling suggested that the action of propofol on GABA receptors in the nucleus reticularis generates a hypersynchronous alpha rhythm (8-13 Hz) with the frontal cortex that blocks sensory input. Hypersynchrony of alpha may block the flexible corticocortical communication required for normal consciousness. A recent animal study identified alpha synchronization between thalamus and medial prefrontal cortex during propofol induction. The potential role of thalamocortical interactions in anesthetic-induced unconsciousness prompts further discussion of the thalamus and its connectivity to the cortex.
Cortical-Subcortical Connectivity
The closely integrated function of the cortex and thalamus suggests that the two can be treated as a single thalamocortical system . The thalamocortical system undergoes state-dependent changes across the sleep-wake cycle, and is thought to play a critical role in consciousness. This role is defined by its ability to integrate the activities of functionally diverse cognitive modules, a property that is critical for subjective experience.
Recent studies using functional magnetic resonance imaging (fMRI) have refined the role of thalamocortical connectivity in anesthesia. One study identified a propofol-induced disruption of connectivity between the thalamus and lateral frontal-parietal networks. Similarly, a study of the specific nuclei (linked to particular sensory modalities) and nonspecific nuclei (linked to integrative functions) found that disrupted connectivity between the nonspecific nuclei and the cortex best accounted for a reduction in the level of consciousness by propofol. Recently, the inhaled anesthetic sevoflurane has been shown to functionally disconnect the thalamus and cortex, especially frontal cortex. Notably, a consistent finding of neuroimaging studies is that the thalamocortical connectivity of primary sensory networks is relatively well preserved despite anesthetic-induced unconsciousness.
The finding of impaired thalamocortical connectivity in association with anesthetic-induced unconsciousness has not been universal. An fMRI study of propofol revealed more profound functional disconnections between the cortex and putamen, a subcortical structure in the basal ganglia. In contrast, thalamic connectivity was relatively well preserved. The potential role of the striatum (composed of the putamen and caudate) in anesthetic-induced unconsciousness has been demonstrated in a study of rats undergoing isoflurane anesthesia. This study was conducted with fMRI and found that the functional connection between the frontal cortex and the basal ganglia was disrupted during general anesthesia. A functional disconnection of association cortex and subcortical structures has also been shown by fMRI in a study of propofol-induced unconsciousness in humans. With improved spatial resolution of 7T fMRI machines and templates for brainstem nuclei, future studies can focus on identifying with greater precision the critical subcortical and cortical interactions or functional disconnections that contribute to anesthetic-induced unconsciousness.
Cortical Connectivity and Dynamics
The last three sections were organized according to a bottom-up approach to consciousness and anesthesia, starting with the brainstem, and then moving to the diencephalon and thalamocortical system. Sleep is clearly generated through such bottom-up mechanisms ; however, anesthetics may suppress the level of consciousness through bottom-up mechanisms and content of consciousness through top-down (i.e., cortical) mechanisms.
Early studies using positron emission tomography (PET) demonstrated regional depression in cortical areas, including lateral and medial frontal-parietal networks. Disruption of fMRI-based functional connectivity in frontal-parietal networks has been demonstrated during anesthetic-induced unconsciousness induced by drugs with distinct molecular mechanisms, including propofol, sevoflurane, and ketamine. However, the cortex is amenable not only to fMRI but also neurophysiological techniques of assessing connectivity, enabling data on anesthetic-induced unconsciousness with improved temporal resolution. EEG can be used to measure functional connectivity (the statistical covariation of the activities of brain regions), and directional or effective connectivity (the presumed causal influence of one brain region on another). Using such techniques applied to EEG (in some cases with concomitant fMRI), disruption of frontal-parietal connectivity and surrogates of information exchange in humans has been consistently observed after administration of a variety of anesthetics with diverse molecular targets. It has been suggested that such disruption of frontal-parietal connectivity, with implications for impaired information transfer of relevance to consciousness, might be a common mediator and proximate cause of anesthetic-induced unconsciousness.
The inhibition of frontal-parietal communication is likely representative of a more global disruption of cortical communication. A study using high-density EEG and transcranial magnetic stimulation revealed an inhibition of cortical-effective connectivity after midazolam-induced unconsciousness. After administration of the benzodiazepine, local cortical activation could be observed at the site of magnetic stimulation, but robust evoked potentials were terminated at less than 100 milliseconds, and cortical communication was limited. Of note, this finding is consistent with findings in NREM sleep. This perturbational approach determined that the complexity of cortical response to a stimulus decreased during sleep, general anesthesia, and disorders of consciousness. The concordant findings across physiologic (sleep) and pharmacologic (anesthesia) states of unconsciousness may reflect a common neurophysiologic mechanism of disrupted cortical connectivity through slow oscillations, which share a number of characteristics in NREM sleep and general anesthesia. A study of three epilepsy patients with intracranial neurophysiologic monitoring revealed that, within 5 seconds of propofol-induced unconsciousness, there was a dramatic increase in the power of slow oscillations. Although single-unit neuronal activity was initially suppressed, it returned to baseline (or above baseline) but was fragmented into highly active and quiescent periods. Neural firing became coupled with the slow oscillation. However, the slow oscillations themselves demonstrated decay in phase coupling with increased distance across the cortex. Thus neuronal spike activity became fragmented into “on” and “off” periods, which became temporally uncoordinated across the cortex. These neurophysiologic conditions dramatically reduce the probability of meaningful corticocortical communication.
More recent trends of analyzing cortical changes during states of unconsciousness take a dynamic approach that reflects not just connectivity configurations but the repertoire of states that can be accessed during general anesthesia. For example, there is a contraction of dynamic repertoire and neural signal diversity during propofol-induced unconsciousness that would preclude the kind of flexibility required for normal conscious experience. Dynamic patterns are impaired during general anesthesia and cortical dynamics are stabilized during general anesthesia. This is likely a discrete, multistage process with distinct dynamic signatures during sedation and general anesthesia ( Fig. 9.3 ). Relating dynamics to connectivity, it appears that the repertoire of functional connectivity patterns in the primate brain becomes more tethered to structural/anatomical connectivity patterns during the anesthetized state. Of note, animal studies suggest that the return of consciousness after general anesthesia is defined by discrete neural states that reconfigure during emergence.

In the next section, we discuss memory, the thread that links conscious experiences together to form the narrative of “self.”
Memory
History and Terminology
Modern understanding of the structure, function, and organization of human memory is deeply informed by the study of amnesia. The most renowned demonstration of this principle occurred in 1957, when Brenda Milner reported the remarkable case of Henry Gustav Molaison (1926–2008), an amnesiac who would become known famously as H.M., and who would represent the single most influential case study in the history of neuroscience. In a procedure intended to treat a refractory seizure disorder, significant portions of the medial temporal lobe (MTL) were removed bilaterally—including the hippocampus, amygdala, and adjacent parahippocampal gyrus. H.M. developed profound and enduring anterograde amnesia, and was unable to establish any new conscious memory, irrespective of the sensory modality. He also developed a temporally graded window of retrograde amnesia, with impaired recall of events occurring during the 3 years preceding his surgery. However, most of his associated cognitive functions—perceptual processing, language, attention, access to semantic knowledge, and capacity to retain small packages of information in constant rehearsal—remained largely or entirely intact. Prior to this report, the prevailing theory—articulated by Canadian neuropsychologist Donald Hebb —was that there was no brain region dedicated to memory function. Instead, memory processes were thought to be distributed and integrated into region-specific perceptual and cognitive functions. For example, the visual attribute of a memory would be wholly served within the striate and extrastriate cortical regions responsible for visual perception. The description of H.M. immediately disproved this model. It became clear that the MTL was a specialized and obligatory structure for the establishment and early maintenance of all conscious memory, irrespective of the modality. The trajectory of memory research was profoundly transformed. Initially, largely independent branches evolved to focus on the structural-functional organization of the MTL ( Fig. 9.4 A ) and the nature of cellular-level neuroplastic processes—the latter notably marked by the description of long-term potentiation (LTP) by Timothy Bliss and Terje Lømo in 1973. Subsequently, emerging technologies enabled the development and investigation of systems-level constructs. Examples include the use of EEG and magnetoencephalography to assess the role of oscillatory phase synchronization in neuronal assemblies, fMRI to identify large-scale networks associated with specific memory functions, and machine learning to classify complex neural network patterns predictive of memory.

Amnesia is the term used to describe one of the cardinal properties of general anesthesia. As understood by most anesthesiologists and laypeople, this description is phenomenological; it states that patients do not recall the events that occur to them while receiving anesthesia. However, this usage confuses a critical mechanistic and semantic distinction. Patients in the deepest states of anesthesia are unable to process and bind perceptual elements into an integrated conscious experience. From the perspective of cognitive neuroscience, the “amnesia” of general anesthesia is not a primary failure of memory, but rather a failure of consciousness. It simply reflects that a conscious experience cannot be reconstructed by memory processes when it does not exist in the first place. Further confusion is added by the frequent use of the term awareness —a synonym for conscious perception—to describe the case in which a patient consciously recalls events occurring during the administration of an anesthetic. This ignores the fundamental principle that memory is functionally dissociable from consciousness. Awareness is necessary for the establishment of memory under anesthesia, but it is not sufficient . Conscious recall can occur only if awareness is accompanied by memory processes in the MTL and elsewhere that establish and preserve a representation that can be reconstructed later.
These important distinctions establish the axiom that patients who form memories while under anesthesia cannot have been truly unconscious; they must have possessed some conscious substrate from which the memory derives. However, the converse inference is not always true: the existence of consciousness will not necessarily lead to the existence of memory if an anesthetic drug is present. Evidence to support this statement is unambiguously encountered in everyday anesthetic practice—patients receiving a small dose of propofol or midazolam who engage in a cogent conversation that they are later unable to recall, or in patients emerging from general anesthesia who follow commands to demonstrate that extubation can proceed safely, yet later cannot recall anything related to this clearly conscious event. Anesthetic drugs must therefore have direct effects on memory processes that are dissociable from those on consciousness—and it is this observation that provides a framework for the systematic study of how anesthetic drugs affect memory.
Organization and Function of Normal Memory
Multiple Memory Systems
When the term memory is used in everyday language, it almost always refers to declarative memory. Declarative memory is the representation of prior events and knowledge that is accessible to consciousness and can be manipulated by attention and executive function. It is the form of memory referred to in the context of anesthetic amnesia.
Further important organizational structure exists within declarative memory. The first is the distinction between episodic and semantic memory. Episodic memory is the recollection of events with a clear spatiotemporal context (as when recalling autobiographical events with a distinct sense of personal experience, time, and place), whereas semantic memory is the capacity to recall and apply meaning, facts, and knowledge without spatiotemporal context (as when recalling that Mount Everest is the tallest mountain in the world without any sense of time and place for the acquisition of that knowledge). The episodic memory system is fast-mapping and highly dependent on the MTL as well as frontal and parietal structures, whereas the semantic memory system is slower and involves distributed cortical regions that closely map to the default mode network, a large-scale system that is active during the resting state and flow of spontaneous cognition. The second organizational structure within episodic memory is the distinction between recollection and familiarity. Recollection involves remembering specific qualitative contextual details about a prior event, whereas in a familiarity judgment, there is a sense that an item has been encountered previously, but beyond that there are no added contextual details. The accepted understanding is that recollection and familiarity arise from distinct processes and neural architecture within the MTL. The perirhinal cortex receives input from sensory association areas and supports familiarity judgments through encoding and retrieval of the identifying qualities of an item (the “what” information). The parahippocampal cortex and entorhinal areas receive input from areas processing spatial information (the “where” information), and support recollection through encoding and retrieval of context. The hippocampus links these two, binding item and context information, and appears necessary for recollection, but plays little or no role in familiarity.
Other forms of memory are dissociable from declarative memory. Findings that amnesiacs could learn a hand-eye coordination skill even while possessing no memory of the task led to the distinction between declarative and procedural memory, which is dependent on the caudate nucleus. Subsequently, memory-impaired patients were also found to have intact priming , which is a nonconscious process in which exposure to a stimulus influences the response to a later stimulus—for example, amnesiacs can name pictures 100 milliseconds faster if they have seen them previously, despite having no declarative memory of the exposure. The neural bases for priming effects are thought to arise within regions serving perceptual and contextual processing of the stimulus, and so vary with the nature of the task. Lastly, a large body of work has extensively elucidated the emotional memory system. The classic experimental model is Pavlovian fear conditioning and its variants, wherein an emotionally neutral conditioned stimulus is paired with an aversive unconditioned stimulus, leading to an involuntary associative physiologic and/or behavioral response to the conditioned stimulus. The circuitry involves convergence of afferent information from all sensory modalities on the lateral nucleus of the amygdala, and the basolateral and central nuclei projecting widely to modulate processes in cortical and subcortical regions (see Fig. 9. 4 B ). With the progressive description of these and other functional distinctions, the framework for understanding memory eventually shifted to models that contained multiple memory systems in the brain, distributed functionally and anatomically.
Working memory refers to the capacity to maintain limited amounts of information in the stream of consciousness, which can be manipulated to perform complex cognitive tasks such as reasoning, comprehension, and learning. The concept evolved from, and has largely replaced, earlier ideas about short-term memory , but the terms should not be used interchangeably, as working memory implies both a short-term memory store and the capacity for manipulation. The most influential current model, first proposed by Baddeley and Hitch in 1974, divides working memory into capacity-limited component subsystems: a phonological loop that maintains information through vocal or subvocal rehearsal, such as when one holds a telephone number in mind; a visuospatial sketchpad , which holds and manipulates spatial, visual, and kinesthetic information; and a central executive , which is responsible for regulating selective attention and inhibition. A fourth subsystem, the episodic buffer , was later added to the model and is responsible for the temporary storage of multidimensional representations and integration with declarative memory.
Working memory has long been understood to not involve the MTL, although the belief that it has no role has recently been questioned in studies of spatial working memory. Notwithstanding, current understanding of working memory suggests that it is served by persistent activity and flexible resource allocation in a distributed cortical network, with a critical hub in the dorsolateral prefrontal cortex (DLPFC) interconnected with parietal cortex, thalamus, caudate, and globus pallidus. The functional and structural distinction between working memory and the MTL does not mean that working memory and declarative memory systems do not interact. Working memory depends on declarative memory representations to provide semantic meaning and context. During working memory tasks, cortical perceptual areas associated with representations of declarative memory become activated and show increased synchrony with prefrontal regions. Reciprocally, encoding of declarative memory is strongly influenced by the nature of processing occurring in working memory, with deeper levels of executive processing resulting in better learning.
The transition of memory from short-term stores through to stable long-term stores is experienced as continuous and was assumed to reflect sequential transfer across systems. However, this model is challenged by rare case examples of patients who have a selective short-term memory deficit but intact declarative memory function, and recent studies have reinforced the view that memories are formed in multiple systems in parallel.
Long-Term Potentiation, Synaptic Tagging, and the Consolidation Model of Memory
The consolidation hypothesis of memory was first proposed by Müller and Pilzecker in 1900. They noted that memory for new information could be disrupted by learning other information shortly after the initial training. This effect, called retroactive interference , is temporally graded such that the susceptibility of the memory is greatest immediately after learning and decreases with time. Müller and Pilzecker proposed that the memory trace must initially exist in a fragile state, but subsequently becomes stable through the process of consolidation. The consolidation hypothesis remains the framework for understanding the temporal course of memory processes and behavior.
For a memory trace to be consolidated, it must of course be created. The term used to describe this process is encoding . Encoding implies that the networks mediating the neural representation of an event as it is experienced do not immediately return to their previous state and are modified in such a way that potentiates reactivation of that representation. The synaptic plasticity and memory hypothesis states that activity-induced synaptic plasticity is both necessary and sufficient for the information storage underlying memory, and within this framework encoding implies that some form of synaptic plasticity has been initiated. However, encoding cannot in itself assure the propagation of a memory trace. Encoding creates the potential for the formation of a long-term memory.
The minimal events that constitute the neural correlates of encoding are incompletely understood. Cellular models demonstrate that functional changes in synaptic strength can occur in the absence of any structural change in dendritic spines. The perpetuation of these initial changes through structural and functional remodeling represents the neural correlate of memory consolidation. The prevailing cellular model for this is LTP, which describes a durable increase in synaptic transmission efficiency following a stimulation protocol. It is now recognized that LTP occurs richly throughout the hippocampus, as well as in other afferent pathways. LTP can be induced by nonphysiologic high-frequency stimulation, but also by stimulation protocols that resemble physiologic activity, the most important being bursts in the theta range (4-8 Hz). This is of notable relevance to memory, as synchronized hippocampal theta oscillations appear critical to successful memory behaviors.
The breadth and depth of literature on the mechanisms of LTP are far too voluminous to summarize here. Nonetheless, certain principles are essential and relevant to anesthesia studies and can be stated succinctly. The induction of most forms of LTP requires activation of postsynaptic N -methyl-D-aspartate (NMDA) receptors, followed by influx of Na + and Ca 2+ . This rise in intracellular Ca 2+ is the critical trigger for LTP. Calcium-calmodulin-dependent kinase II (CaMKII) is then activated and autophosphorylated, leading to cytoskeletal reconfiguration. Activation of several other cell-signaling cascades also contribute to LTP. The terminal expression of LTP is protein synthesis, occurring in both the soma and local dendrites, and resulting in enduring structural changes at the synapse. Protein synthesis inhibitors have been demonstrated to consistently prevent sustained LTP in vitro and learning in vivo.
LTP thus proceeds in two phases: early LTP (E-LTP) is independent of protein synthesis and can be sustained across an interval of minutes to a short number of hours, while late LTP (L-LTP) is dependent on intracellular signaling and protein synthesis and can be sustained across many days. The synaptic tagging and capture hypothesis provides a mechanistic explanation for the observation that the persistence of synaptic potentiation (and memory) is influenced by events surrounding encoding. In this model, synapses activated during E-LTP become tagged via a protein synthesis-independent mechanism. This tag establishes the potential for sustained L-LTP, but for the cascade to continue the tags must capture plasticity-related proteins (PRPs) synthesized in the soma or dendrites in response to neural activity. The synaptic tagging model explains how the thousands of dendrites of a single neuron can be engaged in memory stabilization processes in various states of evolution, because the tagging and PRP capture need not occur as a singular event.
Reconsolidation
A major shift the in understanding of consolidation occurred in 2000, when Nader et al. reported that an old memory for auditory fear conditioning, which would normally not be sensitive to protein inhibitors, can be made newly sensitive if it is retrieved. The implication is that retrieval of a memory renders it transiently plastic, after which it restabilizes. This process is termed reconsolidation , and the period of plasticity is termed the reconsolidation window . The mechanism shares many of the LTP processes that are associated with initial consolidation, but also has features that are quite distinct at both the cellular and systems level. Reconsolidation serves as a modulatory process that enables the strengthening of an existing memory, but it is also clear that it provides a window within which an existing memory is malleable and can be updated with the addition of novel information, or diminished through interruption of restabilization processes. These latter properties have generated significant translational interest because of repeated demonstrations of the ability to modify or even eliminate fear memory in animals through pharmacologic and behavioral interventions that interrupt reconsolidation. Indeed, the erasure of fear memory in humans can be demonstrated through performance of a behavioral extinction procedure during the reconsolidation window.
Phase Synchronization and Coupling
The neurons in assemblies and networks undergo oscillatory activation and inhibition. Phase synchronization of these oscillations supports neural communication by creating transient and dynamic associations between different functional brain regions. Phase synchronization appears to be fundamental to neural plasticity and memory, and numerous studies have demonstrated that dynamics of synchrony during memory tasks can be related to both long-term and working memory performance. Gamma-band (30-100 Hz) synchrony is believed to support an important form of Hebbian plasticity, termed spike-timing-dependent plasticity, in the hippocampus. Both computational models and experimental data provide evidence that rapid changes in the frequency and phase of gamma rhythms modulate this form of plasticity through coordination of presynaptic spikes in assemblies of neurons, effectively classifying which assemblies are interpreted as a single event.
Another property of relevance to memory coding is the coupling of gamma oscillations to the phase of slower, synchronized oscillations within the theta-band (4-8 Hz). Theta oscillations, which are prominent in the hippocampus and entorhinal cortex, undergo phase resetting in response to a stimulus. The phase reset involves widely distributed regions, and is thought to serve as an efficient mechanism for the optimization of interregional communication. Theta phase resetting and synchrony in the hippocampal-entorhinal system are implicated in declarative memory, and in the amygdala-hippocampal system in fear-based memory. Coupling between theta phase and gamma amplitude is connected to declarative memory formation, and, in an elegant model, is proposed to form a code for representing multiple items and spatial representations in an ordered way. Coupling between theta phase and gamma phase enables a more precise temporal coordination of neuronal spikes, and may code for separated representations of multiple items in working memory.
Effects of Anesthetic Drugs on Declarative Memory Function in Humans
There are multiple potential pathways by which anesthetic drugs could interfere with memory and cause amnesia. Most of these cannot be directly assessed in humans, but the plausibility of candidate mechanisms can be indirectly examined by designing experiments that are informed by the characteristics of known memory processes. Studies of anesthetic effects on memory are most informative when conceptualized and designed with reference to robust methods and findings taken from the larger body of memory research.
Behavioral Studies of Retrograde Memory Effects
Notwithstanding some isolated case reports, systematic investigations have found no evidence that anesthetic drugs cause retrograde amnesia in humans. There is no retrograde amnesia associated with induction doses of thiopental, methohexital, or propofol. Adult patients have normal memory for visual stimuli presented 4 minutes before administration of midazolam at doses of 2, 5, and 10 mg. Similarly, memory is normal for word lists learned in the preoperative holding area or operating room immediately before induction. Studies in pediatric patients have shown normal memory for pictures presented immediately before sedation with midazolam, propofol, and dexmedetomidine. In controlled laboratory settings using human volunteers and target-controlled infusions, studies have found no impairment of memory for pictures or words presented before sedative infusions of propofol, midazolam, thiopental, or dexmedetomidine.
There is some evidence from controlled studies that anesthetic drugs may instead lead to the opposite effect, in which memory for material presented prior to drug administration is enhanced—a phenomenon termed retrograde facilitation . In one study using a mildly sedative dose of propofol, memory for words learned immediately prior to drug administration was enhanced throughout the testing period of 24 hours. Similar effects are described for midazolam and other benzodiazepines in the psychopharmacology literature. The mechanistic explanation for retrograde facilitation can be framed by an understanding of retrograde interference —the observation that mental exertion inhibits the consolidation of recently formed memories, with the newest memories being most vulnerable. The induction of new LTP interferes with recently formed LTP and memory performance, even when the tasks are unrelated. However, when the induction of new LTP is blocked by administration of a selective NMDA antagonist after learning, interference with recently formed LTP does not occur, and memory performance improves. A parsimonious explanation for the retrograde facilitation seen with propofol and benzodiazepines is that they similarly modulate the induction of new LTP via a GABA-ergic pathway, freeing consolidation resources that enhance survival of recently formed memory.
The findings of retrograde facilitation and absence of retrograde amnesia are suggestive that the key mechanisms of GABA-ergic anesthetic amnesia, at least in humans, involve very early processes in the consolidation cascade. It is more difficult to rationalize that downstream sequences represent principal targets. Were this the case, the expected effect would be interference with ongoing consolidation of memory for events occurring in the past, creating a window of retrograde amnesia. Nonetheless, an alternate model to the induction hypothesis suggests that GABA-ergic anesthetics exert a direct effect on downstream protein transcription processes, based on evidence from rodent studies.
The absence of pharmacologic retrograde amnesia does not dismiss the repeated finding that, in the clinical setting, a percentage of patients will not recall the immediate preanesthetic period—an effect that increases with age. Although not systematically studied, the complex noradrenergic-mediated effects of acute stress and anxiety on memory may be contributory.
Mathematical Modeling of Anesthetic Amnesia
Mathematical modeling of memory decay has been used to characterize the amnestic effects of multiple intravenous anesthetic drugs, which are accurately described by a two-parameter power decay function:
mt=λt−Ψ
where λ reflects the initial memory strength (an index of encoding), and ψ expresses the rate of decay (an index of consolidation). The drugs are characterized by marked differences in the way they modulate the two coefficients. Propofol is an archetypal amnestic drug—it permits robust encoding of material, but the information undergoes accelerated decay because of a failure of consolidation. In contrast, dexmedetomidine archetypally causes memory impairment because of a failure of information to be strongly encoded but has little or no effect on the subsequent consolidation of memories that are encoded. The benzodiazepine midazolam behaves like propofol at lower doses—selectively causing consolidation failure while leaving encoding intact—but with increasing dose a significant encoding impairment emerges. Thiopental in contrast causes marked encoding failure but demonstrates minimal effect on consolidation. The discrepant patterns observed imply that nonspecific GABA A agonism is not per se sufficient to explain the ability of a drug to cause consolidation failure.
Anesthetic Effects on Attention and Arousal as Modulators of Encoding
The effect of anesthetic drugs on encoding processes is related to the modulation of attention. Selective attention is governed by distinct networks mediating alertness, target orientation, and executive control ; involves modulation of neuronal phase synchrony across short- and long-range connections ; and is necessary for the successful establishment of declarative memory. The dominant effect of most anesthetic drugs on attention is decreased arousal, with the notable exception being the NMDA antagonist ketamine, which has dominant effects on orienting and selection. Thiopental mimics the effect of an attentional challenge task in decreasing activation in the left inferior prefrontal cortex (LIPFC), an effect that is not seen with propofol. When tested at 225 minutes, arousal is predictive of memory for thiopental and dexmedetomidine, while propofol causes a significantly greater loss of memory than would be predicted by modulation of arousal alone. In mathematical modeling of anesthetic amnesia, arousal predicts the coefficient of encoding strength with precision for a range of sedative concentrations of dexmedetomidine, thiopental, midazolam, and propofol. In sum, arousal is predictive of subsequent memory for drugs that impair memory dominantly through causing encoding failure.
Neuroimaging Studies of Cortical Encoding Processes
A limited number of functional neuroimaging studies have evaluated the effect of anesthetics on cortical regional activation during memory encoding. An early PET study investigating sedative doses of propofol using a word memory task identified that activation in LIPFC—a region associated with encoding and subsequent memory for language tasks—was conserved, suggesting that propofol did not block the processes required to support successful encoding. In contrast, activation in DLPFC—a region most associated with executive control functions and cognitive control of motor planning —was decreased. In a subsequent investigation using an auditory depth of processing task, activation of LIPFC was found to be decreased by sedative doses of thiopental, but again was relatively unaffected by propofol. However, other studies have identified decreased activation in encoding areas. An fMRI study of sentence comprehension during light sedation with propofol found that decreased memory for sentences was related to decreased activation in the left inferior frontal gyrus (LIFG, within the LIPFC) and the middle temporal gyrus. Another study using a single-word encoding task showed decreased activation in LIFG at levels of propofol sedation causing memory loss, but also found intact connectivity between LIFG and a number of frontoparietal and temporal regions associated with verbal processing and memory tasks, including the middle temporal gyrus and precuneus.
Some functional neuroimaging studies have used experimental tasks similar to memory encoding tasks while evaluating sedative doses of anesthetic drugs, but have not assessed memory encoding as a primary aim; these are informative, but must be interpreted with caution. An early PET study of sedative doses of midazolam using a tone detection paradigm reported a dose-dependent activation decrease in Brodmann areas 9, 10, and 46, which overlap regions of both DLPFC and LIPFC. An fMRI study of semantic word processing during propofol sedation found a dose-dependent decrease in activation of the LIFG, despite intact behavioral responses. An fMRI study of low-dose dexmedetomidine reported a generalized suppression of bilateral prefrontal activation in Brodmann areas 9 and 10 during an emotional picture memory task, but encoding performance was not specifically analyzed. A recent study using music stimuli found decreased activation in primary and secondary auditory processing areas at sedative doses of dexmedetomidine and midazolam, but not with propofol. Other neuroimaging studies evaluating resting state networks, or passive activation in response to stimuli at nonresponsive levels of sedation, should not be viewed as memory experiments.
Neuroimaging Studies of Medial Temporal Lobe Function
Two methodologically similar studies used event-related fMRI to evaluate MTL activation during low-dose infusions of propofol and dexmedetomidine. The degree of amnesia caused by propofol can be linearly related to decreased activation in the hippocampus bilaterally, an effect that behaviorally corresponded to a failure of consolidation processes. In contrast, dexmedetomidine does not reduce the overall level of hippocampal activity, but the subsequent-memory effect is attenuated, and dynamics of hippocampal activation are less predictive of subsequent memory ; one interpretation is that this reflects a downstream effect from weakened cortical encoding processes. An earlier study of the benzodiazepine lorazepam and the cholinergic antagonist scopolamine found decreased memory to be correlated with decreased activation in the anterior hippocampus, accompanied by decreased activation in the encoding-related regions in the fusiform gyrus and inferior frontal cortex. In a largely exploratory investigation, 0.25% sevoflurane was found to reduce hippocampal activation in response to auditory and visual stimuli, but no memory performance was assessed.
A few studies have evaluated anesthetic effects using electrocorticography and depth electrodes implanted in epileptic patients, but none have been dedicated to memory function. One notable study evaluated the effects of mildly sedative concentrations of propofol on hippocampal spectral coherence and power characteristics at rest. The main finding was a significant increase in hippocampal-rhinal spontaneous coherence in the delta-band, but minimal changes in other bands; cortico-hippocampal coherence was not assessed.
Studies of Cortical Event-Related Potentials
A number of memory-dedicated studies have used the event-related potential (ERP)—a small but stereotypic positive and negative signal fluctuation in the EEG time-locked to a stimulus, and which can be isolated through summation over multiple (usually at least 50) identical trials. ERPs are thought to emerge not because new oscillations are induced, but because of a stimulus-induced phase resetting of ongoing oscillations.
An early ERP study of scopolamine, lorazepam, and diphenhydramine suggested that drug-induced effects on arousal and memory may be electrophysiologically dissociable, although memory was not assessed directly. Changes in the P1N1 and N1P2 early complexes, associated with arousal, were seen for all three drugs. However, changes in later complexes associated with memory, notably the P3 and N2P3, were seen only with scopolamine and lorazepam, and not with diphenhydramine, an antihistamine that causes sedation but not amnesia. This work is extended by a series of studies evaluating intravenous anesthetics and using memory-specific experimental paradigms. In a verbal memory task, propofol amnesia was associated with decreased P300 amplitude at the time of the encoding task. In a subsequent study in which multiple drugs were carefully dosed to equivalent levels of sedation, the true amnestic drugs with memory effects independent of sedation—propofol and midazolam—caused decreased amplitude in the P300 and N2P3 components, with the latter being the best predictor of subsequent memory performance. Across all drugs studied, the N2 latency was related to reaction time, a surrogate measure of sedation.
Another study examined early- and mid-latency ERPs originating from the midline parietal precuneal region (Pz), and related them to coefficients describing the extent of encoding and consolidation failure. Across drugs from multiple classes and at multiple doses, consolidation failure was closely correlated with the P2 amplitude and N2 latency observed at the time of encoding. As the visual P2N2 complex is known to originate from synchronous theta oscillations, one possibility is that a common mechanism underlying the effect of anesthetic drugs on consolidation involves changes in theta oscillations across a distributed cortico-hippocampal network occurring at the time of consolidation induction. Further, the P2N2 and memory decay coefficient were also closely correlated with reaction time, which can be related to interregional synchrony. Unfortunately, no direct measures of cortico-hippocampal theta synchrony have yet been performed in studies of pharmacologic amnesia. Another analysis examined the old-new effect , a robust phenomenon in the parietal ERP that distinguishes the response to initial item exposure from subsequent exposures as a marker of memory strength. Propofol and midazolam caused a significant decrease in the old-new effect at 27 seconds, even though memory performance was maintained, demonstrating again an early marker of impaired memory processes that precedes behavioral detection.
Studies using very different experimental paradigms have evaluated auditory ERPs at multiple concentrations of propofol. Propofol causes a dose-dependent decrease in mismatch negativity and early right anterior negativity, which are elicited in response to specific music signatures and are known to relate to associative memory operations. In contrast, the P1 complex, which derives from primary auditory processing, is not affected even at deep levels of sedation.
Nonhuman Studies of Anesthetic Effects on Memory Processes and Behavior in the Medial Temporal Lobe
Anesthetic Effects on Medial Temporal Lobe Plasticity
GABA-ergic interneurons project within and across subregions of the hippocampus, providing an abundant density of possible targets for anesthetic drugs. In an early study using tetanic stimulation of the Schaffer collateral-commissural pathway, isoflurane blocked the induction of LTP, and long-term depression (LTD) by low-frequency stimulation. These effects were reversed by the addition of the GABA A receptor antagonist picrotoxin, providing strong evidence that the effect of isoflurane on LTP is GABA-ergically mediated. In a similar protocol, amnestic concentrations of sevoflurane caused a failure of LTP which was prevented by the addition of the GABA A antagonist bicuculline. A recent study of sevoflurane exposure in neonatal rats found reduced spine density of apical dendrites, synaptic ultrastructure damage, elevated expression of synaptic vesicle-associated proteins, and inhibition of LTP but not LTD. One study of isoflurane, in which the hippocampus was studied 24 hours following anesthetic exposure, somewhat unexpectedly found improvement in cognitive performance, accompanied by evidence of upregulation of the 2B subunit of the NMDA receptor and enhanced LTP.
A series of investigations have demonstrated that propofol inhibits the induction, but not the maintenance, of LTP, and has no effect on LTD. The effect is blocked by the addition of picrotoxin, implicating a GABA A receptor-mediated mechanism. Notably, one study found that propofol inhibited LTP only at anesthetic, and not at amnestic concentrations. Propofol has also been demonstrated to inhibit a number of component subprocesses of LTP. Propofol reduces expression of activity-related cytoskeleton-associated protein (Arc) in the hippocampus in response to inhibitory avoidance training but does not appear to reduce Arc mRNA, which is notable because it suggests a posttranscriptional mechanism.
The hippocampal GABA A ergic interneuron population is notable for a high density of the α 5 -subunit subtype. Of known significance to memory function, the α 5 -GABA A receptor regulates the induction of LTP in response to a narrow range of frequencies in the theta range. These observations have driven a series of anesthesia studies using α 5 -GABA A -knockout mice. Etomidate blocks LTP measured in CA1 neurons in wild-type mice, but not in α 5 –/– mutants, and behaviorally the α 5 –/– mutants are resistant to the amnestic effects of etomidate, but not to its general anesthetic effects. Further, the effects of etomidate on LTP and memory behaviors are reversed by the addition of L-655,708, which selectively reduces the activity of α 5 -GABA A receptors. The behavioral findings were later replicated with exposure to 1 MAC of isoflurane, with memory deficits observed in wild-type mice, but not in α 5 –/– mutants or in wild types receiving L-655,708. The increase in α 5 -GABA A receptors caused by etomidate and isoflurane does not return to baseline for at least 1 week.
The α 5 -subunit subtype is not exclusive in its association with amnesia. α 4 -GABA A receptors are concentrated in the dentate gyrus and dorsal thalamus, and α 4 knockouts are resistant to the amnestic effects of isoflurane but not to its general anesthetic effects. More equivocally, β 3 -GABA A receptor knockout mice have been reported to be resistant to the amnestic effects of isoflurane, while in a study using β 3 -GABA A receptor knock-in mutants, the β 3 subtype was not significantly associated with amnesia. Similar equivocacy has been found in studies of the α 1 subtype.
The effects of anesthetics on hippocampal theta oscillations in vivo have been studied. One study used a fear-conditioning paradigm to examine amnestic concentration of isoflurane, nitrous oxide, and halothane, and reported that suppression of hippocampal-dependent contextual conditioning was proportionate to slowing of theta peak frequency. Another study also demonstrated that isoflurane caused slowing of theta oscillations in the CA1 neuronal bundle without changing absolute power, while in contrast the nonimmobilizer F6, which causes amnesia without sedation or a loss of motor activity, caused a loss of theta oscillatory power without slowing. Scopolamine causes amnesia at doses associated with acceleration of theta oscillations, which might otherwise be expected to improve learning. However, a significant loss of absolute power is also observed. Together these findings suggest that individual anesthetics may cause amnesia through distinct forms of hippocampal theta disruption.
Human and Nonhuman Studies of Anesthetic Effects on Fear Memory Systems
The Amygdala-Dependent Fear System
The amygdala is a cluster of interconnected nuclei sitting immediately anterior to the hippocampus, with afferent and efferent projections to a wide distribution of cortical and subcortical structures. It is critical to fear learning and memory, and the systematic study of amygdala-dependent classical (Pavlovian) fear conditioning has produced much information regarding the mechanisms and circuitry. The basolateral nucleus of the amygdala (BLA) also modulates the encoding and consolidation of memory in the hippocampus and elsewhere in response to emotion, arousal, and stress. The mechanism is dependent on noradrenergic projections terminating on both α and β receptors within the BLA, and can be triggered by systemic stress mediators—notably glucocorticoids and epinephrine. Amygdalo-hippocampal connectivity occurs via direct and indirect projections, and is dependent on theta oscillatory synchrony. The amygdala contains a number of rational targets for GABA-ergic anesthetic drugs.
Studies of Anesthetic Effects on Fear Systems in Nonhumans
As various forms of fear conditioning represent the dominant experimental method for studying memory in animal models, almost all studies of anesthetic effects on memory offer some insight into their effects on fear systems. Beyond the central conclusion from this sizeable body of literature that GABA-ergic anesthetics in general impair the acquisition of fear memory, a subset of studies are notable for their specific focus on fear circuitry and behavior.
There is substantial evidence that anesthetic effects on fear memory are mediated by GABA-ergic mechanisms in the amygdala. Selective injection of the BLA with midazolam blocks acquisition and stress enhancement of fear memory, while lesions of the BLA block anterograde amnesia for an inhibitory avoidance task in rats receiving diazepam, propofol, and sevoflurane. Further, injection of the BLA with the selective GABA A antagonist bicuculline blocks the effect of propofol and midazolam.
There are certain conditions under which anesthetic drugs can enhance fear memory. When propofol or ketamine is given immediately following conditioning, retention is enhanced, which may represent a form of retrograde facilitation as described earlier, but retention is reduced when dexmedetomidine is similarly administered. In a rat model of PTSD, propofol and ketamine administered immediately after fear learning enhances long-term fear behavior, while dexmedetomidine has a neutral effect. Midazolam given during extinction training, which normally inhibits learned fear expression, blocks the effect of extinction, and also blocks fear-reducing effects when given during preconditioning contextual learning. When sevoflurane is administered at a very low, nonsedating dose (0.11%), fear conditioning is enhanced.
Studies of Anesthetic Effects on Fear Systems in Humans
Three functional neuroimaging studies have directly addressed anesthetic effects on emotional memory. A study using PET and path analysis ( Fig. 9.5 lower panel) demonstrated that the superior memorability that negatively arousing emotional items have over neutral items was unaffected by 0.1% and 0.2% sevoflurane, but lost at 0.25%, and was associated with decreased effective connectivity projecting from the right amygdala to hippocampus, and also from the right nucleus basalis to hippocampus. Further, sevoflurane modulated emotional perception such that items were rated more neutrally. An fMRI study demonstrated that sedative doses of propofol do not reduce activation of the amygdala in response to negatively arousing items, but activation of the hippocampus is markedly attenuated (see Fig. 9.5 upper panel ) and correlates with amnesia and loss of the superior memorability of emotional material. This suggests that cortical and subcortical processes serving emotional interpretative functions and afferent amygdala activation are relatively spared by amnestic levels of propofol, while efferent processes underlying amygdala-dependent modulation of hippocampal plasticity are interrupted. An fMRI study of dexmedetomidine also reported no effect on activation of the amygdala in response to negatively arousing items, but in contrast to propofol, emotional items retained superior memorability, and activation in the left amygdala and hippocampus were correlated with subsequent memory. Taken together, these studies imply that propofol may have a more targeted effect on the amygdalo-hippocampal modulatory axis. One potential explanation is that amygdalo-hippocampal connectivity is markedly interrupted by a loss of theta oscillatory synchrony caused by propofol, whereas α 2A antagonism at the LC causes only limited downstream attenuation of noradrenergic signaling in the BLA.

Clinical Relevance
Amygdala reactivity is of importance in a number of fear-based psychopathologies, including anxiety, phobia, panic disorder, and PTSD. The neurohumoral stress response to surgery and critical illness can cause complex changes in levels of catecholamines, glucocorticoids, and other potential promoters of plasticity in amygdala-dependent fear processes, occurring within a context that frequently contains emotional stressors. It is unclear under what specific conditions elevated (or depressed) levels of these mediators might contribute to long-term changes in neuropsychological functioning, although multiple studies in the critical care setting relate stress exposure, and exogenous administration of catecholamines, to negative long-term outcomes. In a broad surgical population, the incidence of PTSD-complex symptoms related to surgery was reported to be 16% —similar to that observed in victims of violence. The effect—or lack of effect—of anesthetic drugs on plasticity processes in the perioperative or critical care setting thus has a theoretical potential to impact long-term psychological sequelae, both positively and negatively. However, there is presently insufficient human data to inform specific recommendations.
Anesthetic Effects on Implicit Memory Function
Implicit (nondeclarative) memory processes do not axiomatically require a conscious substrate, and are not dependent on classic hippocampal plasticity. Therefore the effect of anesthetic drugs on implicit memory functions may have important distinction from those on declarative memory, and accordingly a number of investigations have sought evidence for implicit processing in unconscious subjects. Many studies have used auditory adaptations of the word stem completion task , or the more rigorous process dissociation procedure , in which subjects are asked to exclude items that have been presented; declarative memory will drive avoidance of the target word, while implicit memory will lead to a familiarity response favoring the target word.
Two early studies in patients undergoing coronary artery and gynecologic procedures showed evidence of implicit learning using word stem completion tasks. Another early study demonstrating implicit recall in cardiac surgery patients correlated performance with preservation of the midlatency auditory evoked potential, and most strongly with the early cortical Pa and Na complexes. Later studies demonstrated implicit memory in both trauma patients and patients undergoing an emergency cesarean section using the process dissociation procedure, and further demonstrated that the degree of processing is related to the bispectral index. However, multiple more recent studies using similar procedures have either failed to show significant implicit priming effects or have been equivocal. Synthesis of these disparate results into a cohesive conclusion is difficult. Although differences in patient populations and anesthetic regimens may be of relevance, methodological challenges may have driven both false positive and false negative results. Investigations evaluating implicit memory processes in pediatric patients under anesthesia have also offered conflicting results. One study reported an increased ability to distinguish a primed animal sound from white noise, but other investigations found no evidence of priming.
References
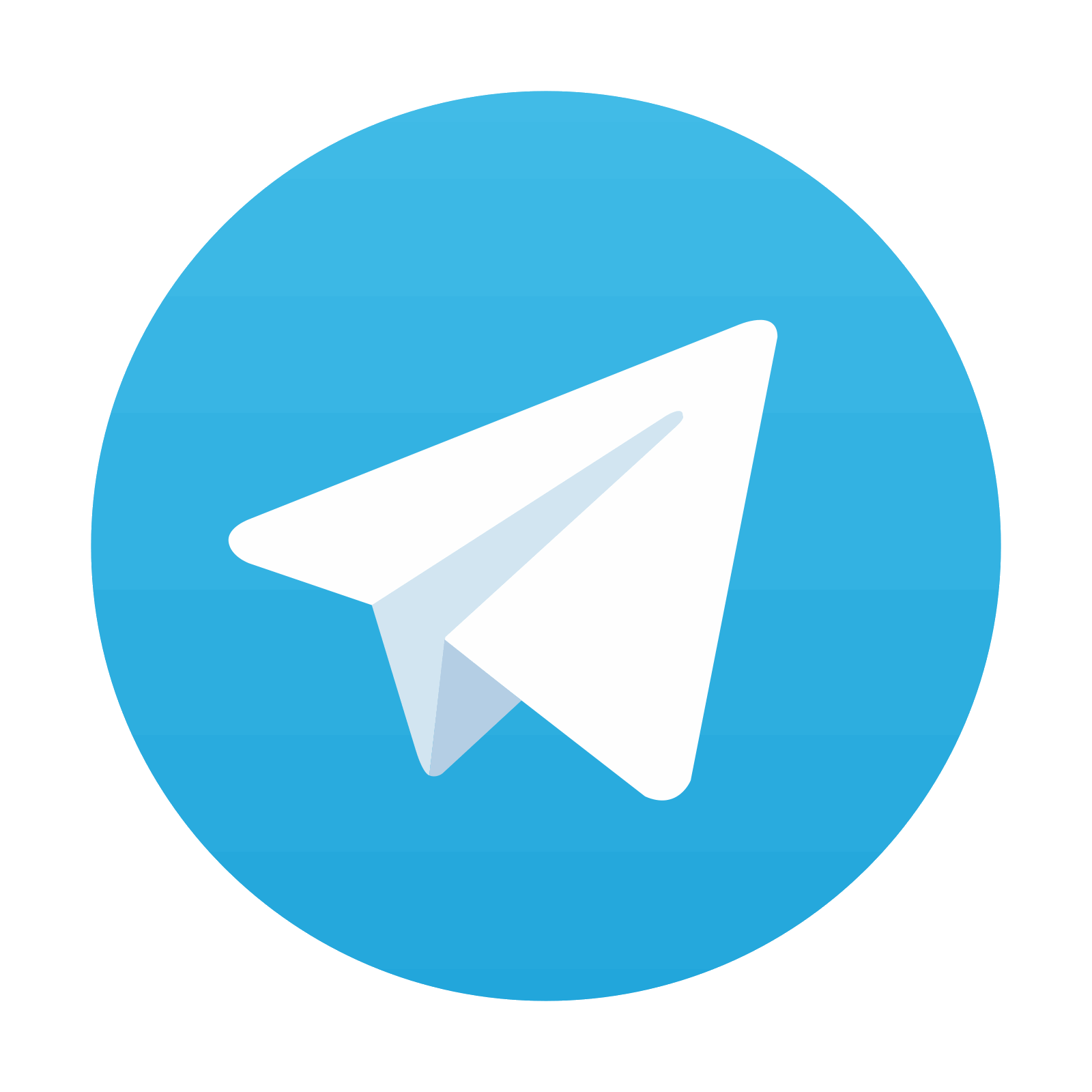
Stay updated, free articles. Join our Telegram channel
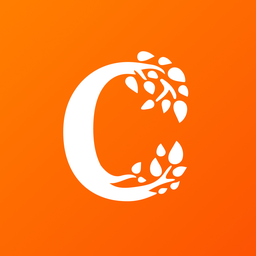
Full access? Get Clinical Tree
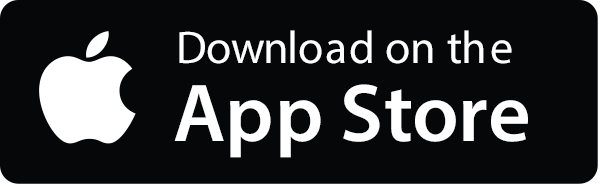
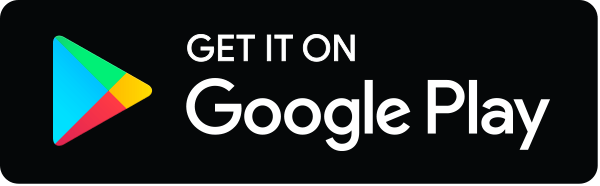