Key Points
- ▪
High altitude or space environments present a number of extreme physiologic challenges that must be overcome in order to survive.
- ▪
Given sufficient time, humans can adapt to both hypobaric hypoxia and microgravity.
- ▪
Lack of adaptation can lead to environment-specific illnesses, such as acute mountain sickness, high-altitude pulmonary edema, decompression illness, or the acute worsening of comorbid conditions.
- ▪
These conditions can rapidly become fatal if not treated appropriately (e.g., with either descent to lower altitudes or returning to the Earth’s surface).
- ▪
Providing critical care or anesthesia in such environments is further complicated by their extreme levels of remoteness.
- ▪
Exploratory missions to such environments depend on the development and vetting of robust and simple health care protocols.
Introduction to Altitude and Explanation of Hypobaric Hypoxia and Its Effect on Physiologic Performance
An estimated 140 million individuals live at altitudes over 2500 m, whereas sojourns to altitude are undertaken by large numbers of individuals each year for leisure, work, and religious reasons. It can be expected that a significant proportion of these individuals will require medical care, either directly related to altitude or for any other reason. As such, there is a clear need for medical practitioners to appreciate the specific environment, physiology, and pathologic conditions at altitude.
At high altitude, a number of environmental changes may be observed. These include decreased temperature, increased ultraviolet exposure and, particularly in mountainous environments, remoteness combined with challenging access and egress, and challenging weather patterns. Overall, the area of primary focus to the critical care and anesthetic practitioner is hypobaric hypoxia and the resultant physiologic changes associated with altitude exposure.
As altitude (i.e., vertical height above sea level) increases, the barometric pressure (P B ) decreases in a nonlinear manner ( Fig. 74.1 ), resulting in a P B at the summit of Mount Everest (8848 m) approximately one third that of sea level. The relative concentrations of gases, including oxygen, remain static but because of the falling P B, the partial pressure of each gas decreases. The resultant hypoxia is of great clinical significance and results in many physiologic changes. These physiologic changes vary with the time course of exposure and a number of long-term adaptations in high-altitude populations have been observed. This chapter considers the high-altitude environment directly, and the consequences of it on clinical practice; however, it should be noted that many consider the hypoxia encountered at altitude to be of translational value to hypoxia at sea level, for example, in critically unwell patients.

Physiologic Responses to Hypoxia/Acclimatization
Cardiovascular Responses
Initial cardiovascular changes occur rapidly. Following the initial ascent, however, more gradual changes may be observed over a period of weeks ( Fig. 74.2 ). On exposure to hypoxia at altitude, peripheral arterial chemoreceptors are stimulated, triggering increased sympathetic activation. The resultant increased heart rate (HR) leads to a rise in cardiac output. A concurrent systemic vasodilation is seen on ascent to altitude. This is antagonized by increased sympathetic tone and the overall effect is one of increased blood pressure (BP), although in the early hours of exposure vasodilation may predominate. After several days, cardiac output returns to baseline but with a lower stroke volume (SV) and higher HR, while BP continues to rise for several weeks.

This pattern of increased HR and reduced SV persists across exercise states at altitude. In both athletes and nonathletes, maximal oxygen consumption (VO 2 max) decreases with increasing altitude, estimated at approximately 1% per 100 m above 1500 m.
Hematologically, a rise in hemoglobin (Hb) concentration is seen in response to altitude exposure. Over the initial days, this is related to a fall in plasma volume of approximately 20%, followed by a rise in red cell production secondary to increased levels of erythropoietin (EPO; which begins to rise within hours of ascent). This change in Hb concentration increases arterial oxygen content (CaO 2 ) and theoretically improves oxygen delivery (DO 2 ) to tissues.
However, this may be compromised because there is a marked increase in hematocrit and plasma viscosity. Viscosity has been observed to increase by 38% in healthy volunteers on ascent to 5800 m. Changes in microcirculation, important for the final phase of DO 2 , have been observed in vivo at altitude with a significant reduction in microcirculatory flow seen in healthy volunteers during altitude exposure, although this does not seem to be directly related to the increase in hematocrit and viscosity. Subsequent studies have shown that this flow is significantly greater in the highly altitude-tolerant Sherpa population, raising the possibility that such microcirculatory adaptation may play an important role in optimizing DO 2 and adaptation to high altitude.
Respiratory Responses
The falling P B at altitude results in a decreased atmospheric partial pressure of oxygen (PO 2 ). This in turn results in reduced alveolar (PAO 2 ) and arterial (PaO 2 ) partial pressures of oxygen. However, the reduction in PAO 2 is accentuated because the saturated vapor pressure of water (P SVP Water ) is unchanged (6.3 kPa) at altitude. Examining the alveolar gas equation, we can see that a proportionally higher saturated water vapor at body temperature ( <SPAN role=presentation tabIndex=0 id=MathJax-Element-1-Frame class=MathJax style="POSITION: relative" data-mathml='PH2O’>PH2OPH2O
P H 2 O
) will result in a decreased PAO 2 .
PAO2=FiO2(PB−PH2O)−(PaCO2/R)
This decrease in PAO 2 leads to the hypoxic ventilatory response (HVR), which is the most important aspect of acclimatization to high altitude. Studies have found that successful climbers demonstrate an improved HVR. The decrease in PaO 2 leads to hypoxic stimulation of peripheral arterial chemoreceptors, which in normoxia are normally suppressed by central chemoreceptors responding to changes in carbon dioxide (CO 2 ). The peripheral arterial chemoreceptors trigger rapid increases in minute volume (V E ) over seconds to minutes. A resulting decrease in PaCO 2 also helps increase the PAO 2 , as explained by the alveolar gas equation, which demonstrates that any fall in PaCO 2 will result in an increased PAO 2.
The resultant hypocapnia following HVR leads to a leftward shift in the oxygen dissociation curve (ODC). This shift improves oxygen uptake at the lungs, but the increase in affinity may have consequences for DO 2 at end organs. A leftward shift and increased oxygen affinity of Hb are key components of the adaptive response in a number of animals who live in hypoxic conditions. However, with altitude exposure in humans, this leftward shift is opposed by an increase in 2,3-diphosphoglycerate, over several days, causing a rightward shift. The final position of the curve varies depending on altitude exposure and level of acclimatization, but in sojourners it appears to equilibrate at almost sea level values, whereas certain groups of high-altitude natives are able to sustain a leftward shift through hyperventilation.
As PaCO 2 decreases, a respiratory alkalosis develops in both arterial blood and cerebral spinal fluid (CSF). This alkalosis initially antagonizes HVR; however, after several hours and continuing for over 2 weeks, the body begins to correct the alkalosis through bicarbonate (HCO 3 − ) excretion, as well as the increased concentrations of protein anions (such as phosphate and albumin). These processes allow the continued increase in V E (and thus improved acclimatization) to progress throughout this period.
The pulmonary circulation is also modulated at altitude. In response to the reduced PAO 2 , hypoxic pulmonary vasoconstriction (HPV) occurs within minutes. The mechanism is a contraction of pulmonary smooth muscle cells. This process is independent of any external regulation and has been demonstrated in the laboratory setting in pulmonary smooth muscle cells completely isolated from all other tissues. The exact mechanism of oxygen sensing is a source of ongoing research, but it is known that the smooth muscle contraction itself, as in other tissues, relies upon a rise in intracellular Ca 2+ . Although the contraction occurs in isolation, it is modulated by both the endothelium and systemic factors such as sympathetic activation. The process is biphasic in nature, with an initial contraction reaching its maximal effect between 2 and 15 minutes. A secondary phase occurs between 30 and 60 minutes, causing further vasoconstriction in sustained hypoxia. This secondary phase appears to be dependent on the presence of endothelial cells.
In contrast to HPV in localized hypoxia (as seen in many pathologies found at sea level, such as pneumonia, atelectasis) where this response is beneficial for correcting ventilation-perfusion (V/Q) mismatch, the global alveolar hypoxia seen at high-altitude HPV can be significantly deleterious. Widespread vasoconstriction increases pulmonary vascular resistance 50% to 300%, with a consequent precipitous pulmonary arterial pressure (PAP) increase that may be responsible for altitude-specific pathologies such as high-altitude pulmonary edema (HAPE) (see later in this chapter). This rise in PAP is further exacerbated by physical exercise with chamber studies reporting a PAP of 54 mm Hg on maximal exercise at a P B equivalent to 7620 m.
Renal and Endocrine Changes
Renal responses play a key role in altitude acclimatization and have long been observed. The hypoxic diuretic response (HDR) was first observed in 1944. It is characterized by both natriuresis and diuresis and is seen in humans and many other mammals.
The earliest phase of the HDR occurs within hours of ascent and is in fact a diuresis alone, with a static fractional sodium clearance. Hypoxia and hypocapnia appear to be the driving factors (although the exact mechanism remains uncertain) and natriuresis soon follows. During the HDR, a total water loss of approximately 3 L is possible during initial exposure and a decrease of approximately 40% plasma volume.
A number of studies suggest that HDR undergoes humoral regulation. The renin-angiotensin-aldosterone system is one of the major systems regulating fluid balance at sea level. Renin and aldosterone promote retention of both water and sodium. However, although renin activity and aldosterone levels are observed to decrease in response to altitude, this response has not been consistently shown to be directly related to the level of natriuresis observed, suggesting there may be a mediation driven by chemoreceptor activation. It is also of note that although the aldosterone exercise response (leading to an increase in plasma aldosterone) remains present at altitude, it appears to be reduced. Indeed, further elements of the hormonal response also appear inconsistent. An increase in serum osmolality is reported with altitude ascent and although this may be expected to produce a concomitant increase in arginine vasopressin (AVP), such a response is often not observed, suggesting a change in AVP regulation at altitude in favor of a diuresis.
The other side of the coin, from a fluid homeostasis perspective, is atrial natriuretic peptide (ANP). It is produced by cardiomyocytes in response to atrial distension and plays an important role in fluid balance; an increase in ANP will induce a natriuresis. It has been observed to increase in response to both pure hypoxia and altitude ascent and may play an important role in the initial diuresis, although any direct causal relationship between ANP levels and the observed diuresis/natriuresis have been questioned.
Although controversy remains around the exact relationship between any hormonal changes and the observed diuresis/natriuresis, a number of studies have reported an association between a picture of increased fluid retention, or hormonal changes promoting fluid retention, and altitude pathologies such as acute mountain sickness (AMS) and HAPE. This suggests that, regardless of the underlying regulation, diuresis remains an important part of successful acclimatization.
In addition to fluid balance, the renal system is also important in mitigating the pH changes generated by the HVR, as discussed previously. Urinary bicarbonate excretion increases over a period of hours to more than 2 weeks, in a process that appears to be unrelated to the natriuresis previously discussed. This reduces blood pH, and may play a role in facilitating the ongoing increase in V E and, therefore, acclimatization to altitude.
Additional endocrine changes may also be observed following altitude exposure. Cortisol, a stress hormone secreted by the adrenal glands, appears to increase at altitude, although exceptions are found in the literature. It may be that this variation is related to duration of exposure, exercise state, and other stress factors, such as coexisting pathology.
As previously discussed, sympathetic activation forms an essential part of the response to acute altitude exposure, which drives many other physiologic changes. As expected, levels of norepinephrine and epinephrine increase, as does nerve fiber activity. However, after prolonged exposure and despite persistently elevated catecholamine levels, maximal HR and response to chronotropic drugs (e.g., isoprenaline) are both reduced, which suggests that a degree of desensitization is also occurring.
Central Nervous System
Several central nervous system (CNS) changes are found at high altitude. Many of the most frequently observed were eloquently described by John West, one of the leading figures in respiratory and high-altitude physiology. On ascending to altitude, unacclimatized lowlanders typically complain that they take longer to get to sleep, wake frequently, often have unpleasant dreams and do not feel refreshed in the morning. The resultant difficulties are not confined to the night because, as a result of poor sleep, people often feel somnolent and fatigued during the following day, their productivity is reduced and they are more liable to make errors.
This quote encapsulates two separate changes observed at altitude: sleep disturbance and cognitive changes.
Sleep disturbance is a frequently occurring symptom, with incidences of up to 65% reported on ascent. Indeed, a network analysis of symptom scores in altitude sojourners identified the largest single cluster of symptoms to be one primarily characterized by sleep disturbance. Subjectively, individuals report reduced general sleep quality, difficulty in falling asleep, frequent wakening, as well as temperature-related discomfort.
At high altitude, the sleep architecture is altered. The duration of light sleep, and non-rapid eye movement (NREM) sleep stages I and II, is increased. Deep sleep and NREM stages III and IV are reduced dramatically, whereas rapid eye movement (REM) sleep duration appears to be more variable in duration. Frequent awakenings are also observed, which appear to be closely linked to another phenomenon seen during sleep at altitude: periodic breathing.
Periodic breathing, as described by John Cheyne and William Stokes in the 19th century and named eponymously for them, has long been observed at altitude. Reports date back as early as 1857 before it was first described fully by Angelo Mosso in 1894. Characterized by periods of crescendo-decrescendo hyperpnea followed by apnea at altitude, periodic breathing is seen most commonly during NREM stages I and II and appears to be largely absent during REM sleep. Periodic breathing may be responsible for the frequent awakenings seen at altitude that often occur at the transition from apnea to hyperpnea.
This breathing pattern is caused largely by hypocapnia, which is itself a result of HVR. Although this hypocapnia during wakefulness does not adversely affect patterns of breathing during sleep, both the rate and depth of respiration can decline, culminating in apnea. During this apnea, the partial pressure of carbon dioxide (PCO 2 ) increases as PO 2 falls, ultimately leading to a return of ventilation with hyperpnea. This theory is supported by the observation that those who demonstrated the most brisk HVR also experienced the highest rates of apnea, along with the efficacy of supplemental oxygen to improve sleep quality. Periodic breathing tends to increase with ascent and may even occur during REM sleep at extremes of altitude. With acclimatization, periodic breathing can be seen to decrease.
The previous quote from John West also alludes to cognitive changes, which are frequently observed at altitude. Studies have demonstrated deficits across a wide range of domains under hypoxic conditions including arithmetic, memory, language, and motor skills. In addition to direct cognitive impairment, there are adverse changes in mood state and even new onset anxiety disorders secondary to high altitude. The exact etiology of these cognitive changes has not been fully elucidated, although fatigue does appear to be correlated with a number of cognitive domains, and as such, sleep disturbance may be contributory.
Gastrointestinal
On ascent to altitude, anorexia is frequently observed but not fully understood. Leptin, a hormone with elevated levels that are associated with satiety, has been hypothesized as playing an important role but the literature is conflicting; elevated, reduced, and unchanged levels have all been reported. Cholecystokinin, another satiety hormone, has been less widely studied but has been found to be elevated, particularly in those with marked anorexia at altitude.
In concert with anorexia, weight loss of both fat and muscle occurs in response to prolonged exposure. This may be related primarily to reduced intake, although a modest increase in basal metabolic rate is observed, and may be partly attributable to sympathetic drive.
Altitude Illness
Several distinct pathologies are observed in association with both acute and chronic altitude exposure. Although each is considered separately here, some share pathologic features. In addition, the hypoxic environment can also affect individuals with other ongoing health considerations, such as comorbidity or pregnancy.
Acute Mountain Sickness
AMS is the most common acute altitude pathology. It is a clinical syndrome of nonspecific symptoms, occurring on ascent to altitude (>2500 m). The onset is usually seen between 4 and 12 hours after ascent.
The prevalence of AMS is closely linked to altitude attained—approximately 25% at moderate altitude (<3000 m) and approximately 50% above 4000 m. Other factors exerting a strong influence are rate of ascent, degree of acclimatization, and individual susceptibility. It is relevant to note that physical fitness appears to confer no risk reduction. Physical exertion at altitude may increase risk, although studies remain conflicted.
The pathophysiology of AMS is not yet fully understood. A number of theories have been suggested and current opinion appears to favor CNS dysfunction as the primary cause. Imaging studies in moderate-severe AMS have suggested some degree of cerebral edema, and increased intracranial pressure (ICP) has also been observed. In one remarkable study by Brian Cummins in 1985, three individuals, including Cummins himself, were fitted with invasive telemetric ICP monitoring. On ascent, two subjects remained well but one developed symptoms of AMS, including headache. This individual was also the only subject to demonstrate a raised ICP. The symptoms were seen on minimal exertion; turning his head increased ICP from 14 to 24 mm Hg, and a press up led to an ICP of 51 mm Hg. This study has never been, and is unlikely to be, replicated but suggests elevated ICP may be pathologically significant and potentially related to reduced intracranial compliance. Cerebral blood flow (CBF) is known to increase transiently on ascent and, although wide interindividual variations have been observed, it may play a role. A unifying hypothesis has been proposed whereby hypoxia and hypoxemia result in increased CBF, which alongside changes in blood-brain barrier permeability, leads to brain swelling. Finally, in those with reduced intracranial compliance, this leads to AMS. This hypothesis, however, remains speculative, with some believing venous outflow may be more significant than intracranial compliance.
The diagnosis of AMS is clinical. The symptoms are variable in presentation but may include headache, nausea, anorexia, dizziness, sleep disturbance, and fatigue. Headache is the most frequently observed indication and remains a mandatory symptom for diagnosis in a number of research tools. Several scoring systems of AMS exist for research purposes. A number of these have been applied to clinical practice, although they are not validated formally for this use. The most widely used, the Lake Louise Score, is ubiquitous in the literature and consists of five simple, self-reported symptom-related questions. This scoring system was revised in 2018 with the removal of sleep disturbance after studies suggested that it is likely to be a separate phenomenon unrelated to AMS ( Table 74.1 ). Laboratory testing and clinical examination in AMS are both unremarkable. Oxygen saturation (SpO 2 ) monitoring has been suggested as a useful tool but although SpO 2 is seen to fall with ascent, there appears to be no independent relationship with AMS.
2018 Lake Louise Acute Mountain Sickness Score | ||
---|---|---|
Symptom | Description | Score |
Headache | ||
None at all | 0 | |
A mild headache | 1 | |
Moderate headache | 2 | |
Severe headache, incapacitating | 3 | |
Gastrointestinal symptoms | ||
Good appetite | 0 | |
Poor appetite or nausea | 1 | |
Moderate nausea or vomiting | 2 | |
Severe nausea and vomiting, incapacitating | 3 | |
Fatigue and/or weakness | ||
Not tired or weak | 0 | |
Mild fatigue/weakness | 1 | |
Moderate fatigue/weakness | 2 | |
Severe fatigue/weakness, incapacitating | 3 | |
Dizziness/light-headedness | ||
No dizziness/light-headedness | 0 | |
Mild dizziness/light-headedness | 1 | |
Moderate dizziness/light-headedness | 2 | |
Severe dizziness/light-headedness, incapacitating | 3 | |
AMS Clinical Functional Score | ||
Overall, if you had AMS symptoms, how did they affect your activities? | ||
Not at all | 0 | |
Symptoms present, but did not force any change in activity or itinerary | 1 | |
My symptoms forced me to stop the ascent or to go down on my own power | 2 | |
Had to be evacuated to a lower altitude | 3 |
Optimal clinical management of AMS begins with prevention. The most significant measure should be a slow ascent, with a limit of 300 m gain in sleeping altitude per day at altitudes above 3000 m generally accepted as best practice, although 600 m is proposed as an alternative. The sleeping altitude is generally accepted to be the most important consideration and the mantra of “climb high, sleep low” can be found widely in the literature. Other nonpharmacologic measures suggested, with some evidence, include: preacclimatization, avoidance of exercise, adequate hydration, and oxygen supplementation. Pharmacologic prophylaxis has been widely studied with acetazolamide the most recommended at an adult dose of 125 mg twice daily. Higher doses of acetazolamide remain efficacious; however, they may be associated with more deleterious effects. Dexamethasone (2 mg every 6 hours or 4 mg every 12 hours) has also been shown to be beneficial, but remains a second-line chemoprophylaxis. Individual risk is highly variable based on both personal (e.g., previous history of AMS or High-Altitude Cerebral Edema [HACE]) and environmental (e.g., rate of ascent, highest altitude attained) factors. Prescribing of prophylactic medications and indeed any recommendations regarding individuals should consider this personalized risk.
When treating AMS, because of the nonspecific nature of the symptoms, consideration must be given to other causes. There may be more severe altitude pathologies such as HACE, or common conditions related to the nature of much travel to altitude such as dehydration, exhaustion, hypothermia, hypoglycemia, or infection. In cases where AMS is diagnosed, then the single most efficacious treatment is descent. This may present other dangers, given the terrain often found in the high altitude environment; however, in severe cases, descent until resolution of symptoms (which typically occurs after a descent of as little as 300 m) remains the gold standard treatment. Measures aimed at correcting hypobaric hypoxia, such as supplemental oxygen and hyperbaric chambers, can offer possible alternatives to descent but in a remote high-altitude setting these treatments present marked logistical challenges, may only offer temporary benefit, and also carry their own risks. Dexamethasone has been shown to be an effective treatment, but it does not aid acclimatization and further ascent is not recommended until the patient is asymptomatic without dexamethasone.
High-Altitude Cerebral Edema
HACE is a severe, life-threatening pathology. It is rare, with a prevalence of 0.28% to 1%. Because of its rarity, there is limited systematic evidence regarding risk factors. However, (not yet conclusively proven) HACE is now considered to be a severe form of AMS and may share the same pathophysiology and risk factors. It is important to note though that while AMS may rapidly progress to HACE if left untreated, HACE may also present suddenly without any preceding symptoms of AMS.
Both animal studies and postmortem examinations have demonstrated gross edema in HACE sufferers, with magnetic resonance imaging (MRI) studies suggesting this is likely vasogenic in nature. In contrast to AMS, in HACE there is evidence of microhemorrhage, primarily within the corpus callosum. The presence of microhemorrhage may indicate venous obstruction as a key pathologic process, which may differentiate AMS from HACE.
HACE may be clinically differentiated from AMS by the presence of ataxia, confusion, psychiatric changes, or altered consciousness that may progress rapidly to coma and death. Investigations offer limited benefit on acute presentation; however, lumbar puncture may reveal elevated ICP, whereas MRI and computed tomography may reveal changes associated with edema.
Prevention of HACE should be guided by the same principles as AMS, with slow ascent, preacclimatization, and pharmacologic strategies as previously described. The diagnosis should consider other possible causes for the observed presentation, but it is important to remember the mantra that any illness at altitude should be treated as altitude-related until proven otherwise. When HACE is diagnosed, its severity should not be underestimated and prompt management is essential. Immediate actions include supplemental oxygen (aiming for an SpO 2 > 90%), administration of dexamethasone (initial dose of 8 mg by mouth, or intravenous or intramuscular injections; and immediately followed by a dose of 4 mg every 6 hours) and, where logistically possible, descent. If descent is not possible, and the airway is adequately protected, then a hyperbaric chamber is an acceptable temporary alternative.
High-Altitude Pulmonary Edema
HAPE is a form of noncardiogenic pulmonary edema that occurs in unacclimatized individuals on ascent to altitude (>2500 m). The condition was first described in South American lowlanders on ascent to altitude in 1955, and subsequently in 1960 by two separate American physicians.
The condition generally occurs within 2 to 5 days of ascent and is more common with greater altitude. It remains relatively uncommon under 3000 m and after more than 1 week at altitude. As with many altitude pathologies, the rate of ascent, altitude, and individual susceptibility are the most significant risk factors for the development of HAPE. There is evidence of other predisposing factors including: male sex, preexisting respiratory infection, and cold temperatures. Cardiorespiratory diseases, including anatomical abnormalities affecting pulmonary blood flow, have also been shown to increase risk of HAPE and it should be noted that altitude dwellers may suffer from “re-entry” HAPE following a sojourn to lower altitudes. The prevalence of HAPE varies greatly depending on the aforementioned risk factors. For example, in the general population prevalence of HAPE is estimated at less than 0.2% when climbing to 4500 m in 4 days. However, for a 1- to 2-day ascent to the same altitude, the prevalence rises dramatically to 6%. In those with a known susceptibility to HAPE, this could reach as high as 60%.
The pathophysiology of HAPE is closely related to HPV and the increase in PAP. Pulmonary hypertension is seen on ascent to altitude prior to the development of HAPE. Individuals who are susceptible to HAPE tend to have a brisk HPV response, and often a relatively blunted HVR which further drives HPV. This accentuated rise in PAP of approximately 60 mm Hg in untreated HAPE on ascent may also be partly related to decreased nitric oxide (NO) bioavailability. The subsequent edema appears to be directly related to this increase in pressure, with bronchoalveolar lavage in early HAPE showing little inflammatory change, while pulmonary capillary pressure exceeds values shown in animal models to cause edema.
Early clinical presentation is most commonly exertional dyspnea, often associated with a dry cough. This results in reduced exercise performance. Dyspnea is normally progressive, leading to dyspnea at rest, while the cough may become productive of pink frothy sputum—with hemoptysis of frank blood being rare. Orthopnea is additionally seen as edema progresses. Symptoms of AMS may coexist, but around 50% of sufferers display none. Examination findings typically include tachycardia and tachypnea. Cyanosis may be present and, although not universal, crepitations are invariably audible on auscultation of the lungs. SpO 2 and blood gas analysis show more profound hypoxia than in healthy controls, while radiographic findings demonstrate patchy edema, generally starting peripherally.
As with AMS, if appropriate steps are taken, the incidence of HAPE can be significantly reduced. Gradual ascent remains the single most effective means of reducing HAPE occurrence. Individuals with a history of HAPE should take additional care and in such cases pharmacologic prophylaxis may be warranted. Nifedipine, a calcium channel blocker, has been shown through randomized control trial and clinical experience to be effective in high-risk individuals, delivering significantly lower PAP and improved prophylaxis of clinical HAPE over a placebo. Other medications, such as salmeterol and tadalafil, have shown promise in clinical trials but clinical experience remains limited. Further research is required, although salmeterol is considered as an adjuvant to nifedipine in high-risk cases. Dexamethasone, which is used widely in AMS/HACE, is not as regularly utilized in HAPE. There is some data supporting its use, but again further study is advised to confirm.
As with AMS/HACE, descent remains the most effective treatment for HAPE. Improvements may be seen after only minimal descent, although descent of 1000 m or until symptoms resolve is advocated. The degree of exertion on descent should be minimized as much as possible to reduce further rise in PAP. Supplemental oxygen and hyperbaric chambers are further measures to consider when descent is not possible. Nifedipine continues to have a role in treatment, with a single unblinded trial showing some clinical improvement and extensive clinical experience supporting its role. Phosphodiesterase inhibitors and dexamethasone may both confer some benefit and case reports of their use exist. It is important to point out that unlike other forms of pulmonary edema, the use of diuretics is not advocated in HAPE, particularly as patients may have concurrent hypovolemia. In the hospital setting it may be possible to manage HAPE without descent using supplemental oxygen and close observation. The use of continuous positive airway pressure has been advocated but remains unreported.
Chronic Mountain Sickness
Chronic mountain sickness (CMS), also known as Monge disease, is a syndrome affecting lifelong altitude dwellers and native populations. First described by Carlos Monge in 1928 in Peru, it is characterized by excessive erythrocytosis (EE), which may lead to pulmonary hypertension, cor pulmonale, and congestive cardiac failure.
CMS prevalence varies widely among high-altitude populations around the world. Tibetans have a low prevalence with 1.2% reported, while with the Han Chinese in the same region the rate is 5.6%. The rates in South America are also generally higher with a prevalence of 4.5% in the general population, and increasing up to 33.7% in miners 60 to 69 years of age. These ethnic variations may be explained by significant differences in adaptation to high altitude. Tibetan natives have been dwelling at altitude for significantly longer than Andean natives and have adapted very differently. Andeans show lower HVR and stronger HPV than Tibetans and even in health show a preponderance for higher Hb than is observed in Tibetans. Other than ethnic variation, there appears to be a direct correlation with altitude; a study in North India observed no cases of CMS below 3000 m, yet a prevalence of 13% above this elevation.
The underlying cause of the EE is not yet fully understood. It is generally accepted that chronic hypoxia, potentially exacerbated by a loss of ventilatory acclimatization leading to central hypoventilation, results in an erythrocytosis. The EE may be mediated by EPO, which is produced in response to hypoxia; however, the correlation between EPO levels, SpO 2 , and Hb is not consistent, suggesting that it is not the sole factor. Recent gene studies have highlighted the potential role of SENP1, a gene involved in EPO regulation. Individuals with CMS SENP1 appear to show a higher transcriptional response to hypoxia and the gene is the subject of ongoing research in this field.
Clinically, CMS may be identified by the excessive erythropoiesis (females Hb ≥19 g/dL; males Hb ≥21 g/dL) and severe hypoxemia. Symptoms of this include headache, dyspnea, fatigue or sleep disturbance, and a burning sensation in the hands and feet. Meanwhile, signs include cyanosis (particularly marked in mucous membranes), finger clubbing, and dilatation of blood vessels. Further investigations may demonstrate pulmonary hypertension and cardiac failure. Of clinical relevance are family history, obesity, and sleep apnea, which are all risk factors for the development of CMS. The gold standard tool for diagnosis is the Qinghai score for CMS. It is important to note that the diagnosis of CMS should only be made in the absence of other systemic disease that may explain hypoxemia, such as chronic airway diseases.
The optimal management of CMS is permanent relocation to a lower altitude; however, CMS will dissipate with the resumption of normoxia. When permanent relocation is not possible, sojourns to lower altitudes may help to stop Hb from hitting excessive levels. Venesection, with or without isovolumetric hemodilution, is used widely in clinical practice. However, supportive literature is of limited quality and there are concerns about a rebound effect on Hb, although these are unproven. In addition, recent evidence suggests that iron deficiency caused by venesection may worsen pulmonary hypertension and advocates the use of iron supplementation to ameliorate this effect. Numerous pharmacologic compounds have been studied with limited success including: ACE inhibitors, ventilatory stimulants, and dopaminergic antagonists. Recently, more focus has been given to acetazolamide with positive results, suggesting it is safe, able to reduce erythrocytosis, and improve pulmonary circulation, although side effects associated with long-term use remain unknown.
Comorbidity at Altitude
The acute altitude pathologies described previously have been largely studied in healthy populations and there is a limited body of evidence to characterize how existing disease may be affected by ascent to altitude. At the same time, travel to remote, high-altitude destinations is becoming easier, and people are living longer with a higher burden of chronic disease ; as a consequence, the challenges of managing chronic disease at altitude are likely to become increasingly relevant.
It is recommended that individuals with significant preexisting disease should consult an experienced altitude physician and undergo a risk assessment before travel. The assessment should consider existing conditions as well as details of the proposed trip, including ascent profile and level of exertion. The conditions most likely of concern at altitude are those affecting the cardiorespiratory system because these may cause additional difficulty in adapting to the hypoxic environment. Consideration should be given to two separate issues: whether chronic disease will predispose individuals to the development of acute altitude pathologies and whether altitude exposure will exacerbate chronic disease.
Ischemic heart disease is a pathology that may be worsened by altitude exposure. Acute altitude exposure increases cardiac output, and thus myocardial oxygen demand, while in the presence of reduced arterial oxygen content. Estimates of myocardial perfusion in healthy subjects suggest that there may be some reduction at altitude, which may be partly ameliorated by acetazolamide. However, several studies have exposed patients with stable coronary artery disease (CAD), who are deemed to be low risk and who have exercised at altitude with no adverse effects. A single study of eight patients with moderate-risk disease demonstrated that compensatory mechanisms of coronary circulation may be exhausted at even moderate altitude. Current recommendations advise cautious ascent to a maximum of 4200 m by low-risk patients, who have had a minimum of 6 months postmyocardial infarction or revascularization before travel. These recommendations also advise moderate-risk patients not to ascend above 2500 m, with only light exercise undertaken, while those at highest risk should avoid altitude entirely. Consideration should be given to acetazolamide, as it may aid coronary perfusion, but this has not been verified in patients with CAD.
Patients with heart failure may pose a significant challenge at altitude, particularly as the condition is often closely linked to other comorbidities. However, studies on patients with stable disease (New York Heart Association Class II-IV) have demonstrated moderate altitude may be tolerated without significant compromise, although those with the most severe disease were significantly limited in their exercise tolerance. Medications used to manage heart failure should also be closely reviewed. Use of diuretics must be monitored, as fluid status is likely to alter at altitude and particular care should be paid to concomitant use of acetazolamide. Studies suggest that selective β1 receptor antagonists may improve exercise performance when compared to nonselective beta blockers and are advised where practical. In summary, those with the most severe disease should abstain from travel to altitude whereas those with mild to moderate disease should proceed with caution.
In the presence of the hypobaric hypoxia found at altitude, there is concern for exacerbation of respiratory disease. Counterintuitively, however, observational data suggest that asthma is less common, and less severe, in those living at altitude. One likely reason for this is the reduced population of dust mites at altitude secondary to the cold, dry, and hypoxic conditions that result in a significant improvement in many pathologic features of asthma. However, such studies do not provide evidence regarding acute altitude exposure. There have been a number of observational studies exploring acute altitude exposure with conflicting results for physiologic measurements (e.g., peak expiratory flow, forced expiratory volume in 1 second [FEV1]), but all have demonstrated safe travel for patients with mild asthma to altitudes of up to 6410 m. Caution is advised, however, as upper airway infections, particularly in remote environments, remain a risk for asthmatic patients. Hence, while travel to altitude is safe for well-controlled asthmatics, adequate supplies of rescue inhalers and oral steroids are advised. Measures may also be taken to limit cold, dry air exposure, which often exacerbates asthma. These can be as simple as covering the nose and mouth during outdoor exposure. Those with poorly controlled or severe asthma are at high risk because of the remoteness of the environment and variability of medical facilities, and travel should be avoided where possible in this group.
Chronic obstructive pulmonary disease (COPD) poses a particular challenge at altitude. Studies of altitude dwellers with COPD demonstrate increased mortality and cor pulmonale. A number of studies simulating altitude as a model for commercial air flight have shown marked hypoxemia in response to moderately simulated altitude. A similar drop in PaO 2 was observed in mild to moderate COPD sufferers on ascent to 1920 m, with a mean PaO 2 fall from 8.8 kPa at sea level to 6.9 kPa. However, no adverse clinical events were reported in these studies and, given the chronic nature of the hypoxia, there is likely to be some degree of preexisting adaptation. Particular care must be taken in COPD patients with pulmonary hypertension. As previously discussed, the pathophysiology of HAPE indicates that preexisting pulmonary hypertension may increase susceptibility to HAPE. When assessing risk of hypoxemia at altitude, the use of formulae derived to predict PaO 2 during flight may be helpful. In general, there are limited data on the safety of travel to altitudes for patients with COPD, and no data for their travel above 3048 m. Patients with COPD, and in particular those with pulmonary hypertension, should seek medical advice prior to traveling to high altitude.
Special Situations at Altitude
Children
Children represent an understudied group at altitude. While many of them either live at or sojourn to higher elevations, comparatively few studies have examined this. Children born and living at altitude are at increased risk of hypoxemia either secondary to acute pathology or congenital abnormalities. However, the risk and responses to living at altitude vary greatly among different ethnic populations as a result of the variations in adaptation as previously discussed.
Studies on acute exposure to altitude suggest that children show similar patterns of acclimatization as adults. Altitude pathologies meanwhile present a significant problem in children. A study in Colorado at 2835 m estimated a 28% prevalence of AMS in children. However, the diagnosis of AMS remains problematic in children, as many symptoms of AMS may be associated with any travel in children. The same holds true for more serious altitude pathologies of HAPE and HACE, which may be overlooked in children (especially of younger age). As a result, extreme caution and descent is advised when a child becomes unwell at altitudes above 2500 m. Pharmacotherapy is less widely advocated in children, particularly for mild cases of AMS. In severe cases or where HAPE develops, there may be a place for weight-appropriate doses of acetazolamide or nifedipine.
Pregnancy
Maternal hypoxia may develop as a consequence of travel to high altitude (>1800 m) with obstetric guidelines identifying this as potentially hazardous for the fetus, although evidence suggests a number of pregnant women will travel to altitude. There are limited data available on this subject, with only a small number of studies exploring on sojourners to altitude. There is evidence of an increased rate of neonatal intensive care admission and oxygen administration at birth in infants of altitude residents. In addition, multiple population studies demonstrate a significant reduction in birth weight that is proportional to altitude gain above 2000 m. This is not due to increased prematurity but rather intrauterine growth restriction (IUGR). The effect of altitude on birthweight in these populations varies among different populations. It is more pronounced in recently arrived populations, such as the Han Chinese and Europeans, and least pronounced in groups such as Tibetans, who have resided at altitude for much longer. This suggests any results derived from sojourners or permanent residents are unlikely to be entirely transferable.
The limited number of physiologic studies on the fetal effects of maternal hypoxia suggest changes in the placental villous membrane facilitate increased gas exchange. With these changes, the fetuses of women exposed to short administrations of 10% oxygen show no signs of distress with no significant change in fetal heart rate, HR variability, or Doppler velocimetry in the umbilical artery. However, studies on altitude dwellers have demonstrated a reduction in the pregnancy-associated increase in uterine artery blood flow, which may account for IUGR.
Additional research is required in this field and the paucity of evidence makes any firm recommendation challenging. There are clear physiologic sequelae for both mother and fetus in response to altitude, which in the chronically hypoxic mother appear deleterious. Consequently, any travel to altitude during pregnancy must be with caution, although conclusive data for sojourners are unavailable.
Anesthesia at Altitude
General Principles
Most surgical facilities are not situated at extremes of altitude. However, a number of centers perform operations at high altitude, while at any altitude there may be an emergent need for general anesthesia. Practice under these conditions is guided largely by clinical experience, the setting, and select published case reports.
The anesthetic practitioner at altitude should be aware of the physiologic and pathologic changes, discussed previously, which may affect individuals. They should endeavor to assess on an individual basis how these changes can impact each patient. Consideration should be given to the altitude at which anesthesia is performed, the level of acclimatization of the individual, and any concomitant pathology. When logistically feasible, it may be advisable, particularly in unacclimatized individuals, to descend before administration of anesthesia. At extremes of altitude, the risks associated with anesthesia are only outweighed by life- or limb-saving procedures.
It is also important to establish the environment in which anesthetisa will take place. The operating environment in a hospital with formal operating theaters and associated equipment is very different to emergency surgery undertaken in a remote and rural setting, regardless of altitude.
Several aspects require particular consideration, the most obvious of which is the increased risk of perioperative hypoxia. Medications used during anesthesia, notably opiates, depress both the tachycardia and tachypnea associated with the physiologic response to altitude. Slowed recovery from anesthesia has been reported, as has increased sensitivity to anesthetic medications. These factors combine to create a prolonged period where an individual may not be able to enact the full range of compensatory mechanisms to tolerate hypobaric hypoxia. Some of these consequences may be reversed by the administration of supplemental oxygen and certainly oxygen therapy at altitude is advised when possible. Care with analgesic agents in the postoperative period is necessary to ensure ventilation and oxygenation is not compromised; analgesia-induced respiratory depression was implicated in the death of a Sherpa who underwent a debridement at 4300 m.
The second area of particular note in the perioperative period are reports of increased bleeding and challenging hemostasis during surgery at altitude. This is attributed to increased venous pressure and increased capillary density and, while not conclusively proven, still warrants consideration.
Anesthetic Equipment
The most routinely considered implication of high altitude on anesthetic equipment is in relation to anesthetic vaporizers. As variable bypass vaporizers rely on the saturated vapor pressure of anesthetic agents, the partial pressure of anesthetic gas delivered remains constant regardless of altitude, although the Fi AA will rise. This should in theory mean the same clinical effect. Chamber-based studies on vaporizers have confirmed a constant partial pressure delivery; however, several caveats to this exist. First, desflurane vaporizers operate by using a measured flow principle. Such vaporizers will deliver a constant Fi AA and, therefore, in hypobaria will deliver a reduced partial pressure. Second, consideration must be given to the monitoring and targeted dose of anesthetic gas. The minimum alveolar concentration is often used to guide dosing of anesthetics but it is not appropriate for use at altitude. Instead, practitioners should consider their administration as a partial pressure rather than a fraction, or concentration, as it is the partial pressure that determines depth of anesthesia. This is most apparent when considering nitrous oxide, which when delivered at a given concentration is less effective at altitude, proportional to the drop in partial pressure.
This recommendation regarding the use of partial pressure should also be considered for all forms of gas monitoring. Modern anesthetic machines may interchangeably state partial pressure or concentrations for gas monitoring. However, the analyzers themselves physically detect partial pressure, not concentration. The reported concentration is derived mathematically and, for example, an oxygen analyzer set to measure 21% in air at sea level will read 17.4% in air at 1500 m, unless recalibrated. Once again, the physiologic significance is in the partial pressure of oxygen. Therefore, direct measurement of partial pressure allows greater understanding of the physiologic effect of the gas mixture being delivered to the anesthetized patient.
Flowmeters are also affected at altitude. Flowmeters rely on the density of gas to measure flow. Studies examining the floating flowmeters showed that with reducing P B , and therefore density of gas, these flowmeters were liable to be under-read. The percentage of error observed was not constant but reached a peak error of 21%, making adjustments challenging. Studies have not been conducted to observe the impact of high altitude on flowmeters found in more modern anesthetic machines such as hot wire anemometers. Caution is advised when examining readings from flowmeters and where possible gas analyzers directly measuring partial pressure should be used to corroborate.
General Anesthesia
Several considerations for anesthesia at altitude have already been discussed, including perioperative hypoxia and the effects of anesthetic drugs on mechanisms of acclimatization. As such, when considering anesthetic agents, the maintenance of these mechanisms, including tachycardia and hyperpnea, may be desirable. This is particularly relevant in the austere environment where supplemental oxygen may not be available. In such environments, the use of ketamine has been advocated in both emergency and elective settings. These reports have varied in their approach, with some using entirely ketamine anesthesia, whereas others have supplemented with inhalational anesthesia or benzodiazepines. Without supplemental oxygen, some degree of desaturation may be observed in the spontaneously breathing patient, but this was felt acceptable and could be managed with monitoring and simple airway maneuvers. However, total apnea may be seen with smaller doses of ketamine than would be expected at sea level, with a report of deep general anesthesia and apnea at a dose of only 0.5 mg/kg; however, other studies have successfully used higher doses without any adverse outcome. Further caution is advised when using ketamine, as it may increase pulmonary vasoconstriction, with a single study at 1600 m suggesting certain susceptible patients may develop increased pulmonary vascular resistance with ketamine use. This may make it an undesirable agent for use in patients with active, or high risk of developing, HAPE.
Any anesthetic at altitude, particularly emergency remote surgery, remains comparatively high risk because there are limited data on which to base practice. There is some literature suggesting that ketamine may be used safely (either alone or in combination). Adequate monitoring, airway equpment, and appropriately trained practitioners are advised, alongside a careful risk-benefit analysis and consideration of alternative courses of action.
Regional Anesthesia
Spinal anesthesia may be desirable at altitude, as it allows the individual to maintain his or her own ventilatory parameters and reduces much of the risk surrounding perioperative hypoxia. Reports of very high rates of postdural puncture headache (PDPH) do exist; however, these predate the use of modern spinal needles with narrower gauges and pencil-point needles now routinely used in modern practice. A single study examining spinal anesthesia at moderate altitude (1890 m) found that at altitude spinal blocks took longer to perform, and block duration was shorter than at sea level. They also observed a higher rate of PDPH (7.14 vs. 2.85%; P < .05) at altitude. Despite these limitations, spinal anesthesia provided adequate analgesia for lower extremity surgery in all patients and no significant complications were reported. Based on this limited evidence, spinal anesthesia may be the first choice for such procedures at high altitude but further study is advised.
Application to Flight, Including Air Transport of Critically Unwell Patients
The contents of this chapter remain relevant when considering air travel. Cabin pressure in commercial air travel is regulated but studies have shown peak cabin altitudes do vary, with an average value of 1933 m and the highest recorded being 2606 m. The physiologic changes are equivalent to those experienced by visitors to high altitude but with the caveat that ascent happens much more rapidly with less time to acclimatize. A small drop in SpO 2 may be observed, and symptoms of AMS have been reported. Despite this, air travel is generally physiologically well tolerated. Greater concern may be associated with prolonged immobility and the risk of thromboembolic phenomena. In contrast, patients with significant comorbidity may not tolerate air travel because of the rapid altitude gain and relative hypoxia. Extensive guidelines exist to guide the decision on flying with health conditions. In the rare event of cabin decompression, risks are considerably higher. In addition to acute hypoxia, which at altitudes above 13,716 m may induce unconsciousness in as little as 15 to 20 seconds, there are dangers posed by sudden air movement, cold, and debris.
The transfer of an unwell patient is a significant undertaking that relies on careful planning, adequate infrastructure and equipment, and a thorough understanding of the patient’s physiology and pathology. In addition to hypoxia, there should be an awareness for gas expansion within body cavities. This expansion may affect pathologic processes, such as pneumocephalus, pneumothorax, or intestinal gas, which is particularly significant in cases such as volvulus. The presence of any of these pathologic processes is a relative contraindication for aeromedical evacuation, but the decision must be ultimately be balanced against the need for ongoing treatment not available at the patient’s location. Gas expansion also changes the volume of air within medical devices, such as endotracheal cuffs. Ascent to 2500 m from sea level increases the volume in an endotracheal cuff by approximately 35%, which should be considered and accounted for both on ascent and descent. There are additional working challenges related to the enclosed space and moving of patients. A large cohort (19,228) of aeromedical evacuations in Canada reports a critical event on 5.1% of evacuations at a rate of 1 per 12.6 hours of transport time. Critical events were more likely to occur in older patients, those with greater hemodynamic instability, and those requiring assisted ventilation. The most common critical event was hemodynamic deterioration and the need for intubation was the most common major resuscitative procedure required. The mortality in the cohort remained very low at 0.1%. These results demonstrate that with appropriately trained practitioners, aeromedical evacuation can be performed safely and with great benefit to patients.
Space Medicine
Atmospheric pressure decreases exponentially with increasing distance from the Earth’s surface and becomes progressively hostile to human survival. At the cruising altitude of commercial airliners (approximately 39,000 feet), the atmospheric pressure outside the aircraft is only approximately 20% of the pressure at sea level. The International Aeronautics Federation defines the boundary of outer space, where the Earth’s atmosphere stops and space starts, as an altitude of 100 km above the Earth’s surface. However, at any particular time, astronauts on board the International Space Station (ISS) are 350 to 450 km above the Earth’s surface as they circle our planet at velocities of up to 17,500 mph. Yet, these extreme altitudes are still considered to be relatively low Earth orbits and future astronauts will travel much farther away from the protective environment of the Earth.
Introduction to Space Exploration and Medicine
On November 5, 2015, the National Aeronautics and Space Administration (NASA) announced it was looking for astronauts for long-duration space flights, including extended missions on the ISS and possible journeys to Mars. In recent times, both the United States and Russia have announced their intentions to construct a new spaceport on the moon and to use this lunar base as a stepping stone to send their own astronauts to Mars. Private billionaire entrepreneurs (such as Richard Branson and Elon Musk) have outlined similar visions and promised to revolutionize air travel by transporting passengers using rockets traveling through space instead of traditional airplanes traveling through the earth’s atmosphere. An international “space race” is undoubtedly already well underway.
Technology, skills, and knowledge have all developed rapidly since humans first entered space in 1961 when Yuri Gagarin orbited the Earth for the first time during a flight lasting less than 2 hours in total. Yet since then, only 12 men have ever walked on the moon and over four decades have passed since the last Apollo mission (Apollo 17) left Earth. A future that includes successfully leaving low Earth orbit again and sending astronauts on more distant missions, as well as making spaceflight safe for commercial purposes, poses many challenges, including how best to identify and manage any potential health issues among future crewmembers and passengers.
Risk of Disease Among Astronauts During Space Travel
Although the absolute chance of a medical issue arising during spaceflight is low, the risk remains significant. Current conceptual plans for future missions to Mars from NASA predict crewed missions lasting up to 1100 days (approximately 3 years). Given the rate of emergencies among the general population is around 0.06 events per year, a crew of 6 astronauts would expect to encounter at least one medical emergency during a 3-year space mission (0.06 × 6 × 3 = 1.08 events).
Astronauts can be affected by illness or disease just as any other human. For example, concern about possible exposure to the German measles virus meant that NASA Astronaut Thomas K. Mattingly was famously removed from the crew of the ill-fated Apollo 13 mission just 72 hours prior to launch (Mattingly never actually developed measles and went on to successfully fly future missions including both Apollo 16 and STS4). However, the unique environmental conditions associated with spaceflight can also modify an astronaut’s susceptibility to such illnesses. Identifying these effects remains an active focus of research, such as the Longitudinal Study of Astronaut Health that NASA has been operating since 1992. This cohort study is generating data about all-cause astronaut mortality and morbidity, such as the reassuring finding that cancer mortality among astronauts is not significantly increased (as might be expected from increased exposure to harmful doses of radiation, as later discussed), suggesting the many preventative measures employed to mitigate these risks during spaceflight appear to be effective.
Similarly, the spaceflight environment can also cause a number of specific health concerns to astronauts that are not normally experienced by the general population and which can affect the physiologic and psychologic performance of crewmembers. One survey of astronauts returning from 79 U.S. space shuttle missions showed that as many as 94% of crewmembers took some form of medication at some stage during the flight and often to treat conditions not normally expected among similarly physically fit cohorts on Earth. These conditions included space motion sickness (SMS), sleeplessness, headache, or backache.
The Environmental Challenges Posed by Spaceflight
Extreme Accelerations/Decelerations
Takeoff and landing (including re-entry to the Earth’s atmosphere) are two of the most important and dangerous periods of any spaceflight. Every human fatality during spaceflight to date (Challenger, Columbia, Soyuz 1, and T11) has occurred during one of these critical periods. Astronauts routinely experience extreme accelerative forces of around 4 to 5 G during takeoff and re-entry but these can be much higher; Yuri Gagarin remained conscious while experiencing forces of around 8 G during re-entry in his spacecraft Vostock 1 in 1961, and the Apollo astronauts typically experienced landing forces of around 17 G and tolerated them remarkably well. Current ISS crews returning in Russian Mir spacecraft use seats with specially designed shock absorbers and liners to decrease these forces by 20% to 30% and bring them down below 22G.
These accelerative and decelerative forces are more than capable of dislodging incorrectly stowed items of equipment (causing trauma from the impact of flying objects or hard landings a real hazard), and also generating extreme temperatures from friction with the surrounding air. During re-entry, the outside of the U.S. space shuttle reached temperatures in excess of 15,000°C. This was high enough to ionize air and remove electrons from the outermost atoms on the protective heat tiles around the Shuttle spacecraft surface, generating significant amounts of electromagnetic disturbance that prevented any radio contact with the on-board crew for 17 minutes. On February 1, 2013, damage to one of the heat tiles during takeoff led to a weakness in the Space Shuttle Columbia’s critical heat-protective layer, leaving the vehicle unable to withstand these extreme temperatures on its return to Earth and causing the vehicle to burn up on re-entry, resulting in the loss of all seven crewmembers on board.
Radiation
Solar radiation exposure (i.e., galactic cosmic rays, high-energy photons, high-energy and charge (HZE) neutrons and nuclei, and solar particle events) has always posed a major health concern for astronauts. These risks of both acute (mission-critical) and chronic (post-mission) radiation-related exposure are only going to increase on longer-term exploratory missions deeper into space. Crewmembers have not completely left the protection of the Earth’s magnetic field (i.e., gone beyond the Van Allen belts) since the Apollo missions, but crewmembers on any future mission to either the moon or to Mars will be at risk of direct exposure to full solar radiation doses for the first time in over 40 years and currently no adequate shielding strategy has been fully developed and tested. Chronic exposures of less than 1 sievert (Sv) per year are still thought to have long-term effects on tumor rates; acute exposures of 0.5 to 1.0 Sv could be sufficient to cause symptoms of acute radiation exposure. Some estimates predict exposure rates could almost nearly reach such levels during a 940-day Mars mission. Other estimates have even projected radiation exposure levels during a simulated Mars mission, that would for the first time even exceed the strict occupational career limit NASA currently employs (i.e., upper confidence interval [CI] for risk of exposure-induced death [REID] of 3%), using the recommendations by the National Council on Radiation Protection. During a 1-year deep-space mission at minimum average solar radiation levels, the total risk of exposure-induced cancer in a 45-year-old male who has never smoked is predicted to be approximately 2% (CI 0.53%-7.84%), with the risk anticipated to be highest for lung cancer followed by colorectal cancers. However, our overall understanding of the long-term health risks from radiation exposure during spaceflight is still relatively limited and marked differences between current predictions and previously observed effects remain. Besides malignancy, other chronic radiation exposure health risks have been noted among astronauts. For example, increased rates of cataract formation have been reported in those exposed to greater than 8 millisievert (mSv) of radiation after a 20-day space mission, , reduced fertility (and/or increased numbers of birth defects in animal studies), and a slightly (but not statistically significant) increased risk of developing clinical thyroid disease. Initially, the CNS was thought to be relatively resistant to the effects of radiation; however, widespread CNS changes have been reported including memory impairment, changing synapse plasticity, impaired executive function, and cognitive dysfunction. Moreover, many of these effects may be even further compounded by the significant neuropsychologic challenges of spaceflight.
Isolation, Confinement, Sleep Disturbance, and other Psychological Challenges
Traveling at over 17,000 km/h, the crew of the ISS travel completely around the Earth—what we usually define as a typical “day”—every 90 minutes. This means that in every 24-hour period, the ISS crew experience no fewer than 16 sunrises and 16 sunsets; in other words, crewmembers could easily see 365 sunrises (a whole year’s worth) in just a 23-day space mission. The sleep-wake cycle is closely linked to biologic circadian rhythms regulated by the solar light, but astronauts also face many other challenges to sleeping comfortably, such as constant noise, physical discomfort (astronauts usually sleep in sleeping bags fixed tightly to the walls of the space craft), and hypercapnia. Sleep deprivation and fatigue are common complaints among crewmembers, with studies suggesting that on average astronauts experienced just 5.96 hours (SD 0.56) sleep during space shuttle missions, 6.09 hours (SD 0.67) during ISS missions, and less than 6.5 hours during their 3-month pre-launch period. Similar levels of sleep disturbance have been reported to impair cognitive performance and worsen long-term health outcomes. The constant stresses posed by extreme isolation and prolonged periods of confinement can also increase sleep disturbances and affect individual and team behavioral performances. Interestingly, though, these effects seem much more variable between different individuals and would also appear to be trainable. In the Mars 500 study, 6 males were confined for 520 days in a 550 m 3 chamber simulating a high-fidelity Mars mission. One crewmember reported depressive symptoms throughout 93% of the mission, whereas two other crewmembers had no symptoms of psychologic distress at any point in the study. Similarly, after reviewing how their astronauts performed during missions on the ISS, all crewmembers participating in the study displayed unusually high levels of self-awareness and were generally positive about their time on the ISS, leading NASA to conclude that their training programs regarding interpersonal relationships, teamwork, and psychologic support were effective.
Decompression and Changing Oxygen Concentrations
Foreign space objects pose a constant hazard to any manned space vehicle, as any penetrating impact could cause sudden depressurization of the internal cabin environment. NASA estimates that there are now more than 21,000 orbital debris objects (usually human made objects with no further usable purpose or the remains of such objects) in the Earth’s atmosphere, and approximately 200 kg of meteorite material (rocky material passing through the Earth’s atmosphere while orbiting the sun) is likely to be within 2000 km of the Earth surface at any particular time. Sudden loss of the crew’s artificial environment can cause acute hypoxia, decompression sickness (DCS), and ultimately death if the problem is not rapidly resolved or contained. According to the Federal Aviation Administration, the time of useful consciousness (i.e., the amount of time a person can effectively or adequately perform flight duties with an insufficient supply of oxygen) after rapid decompression at 50,000 feet is just 5 seconds.
DCS (commonly known as “the bends”) arises when gases usually dissolved are forced out of solution by sudden pressure changes to form generalized bubbles (a process called ebullism, body fluids boiling as a result of ambient pressure being less than the saturated vapor pressure of water). Soviet Cosmonaut Alexei Leonov reportedly gave himself DCS in 1965 as he finished making the world’s first space-walk—he needed to reduce the size of his malfunctioning spacesuit in order to fit back through the airlock door into his space craft! Space suit design has since improved massively but the dangers associated with performing extravehicular activities (EVAs) remain significant.
American and Russian spacesuits maintain an internal environment of 100% oxygen at either 30 or 40 kPa, respectively. It is a major engineering challenge to build flexible suits durable enough to withhold higher pressures against the vacuum of space. Astronauts undergo pre-EVA procedures before leaving the main spacecraft to minimize the risk of developing DCS during a particular EVA, but despite being relatively time-consuming in spaceflight terms, these pre-EVA activities represent extremely rapid decompression times. Atmospheric pressures between 30 and 40 kPa are equivalent to standing on the summit of Mount Everest at 8848 m. While most climbers would typically take around 60 to 70 days to reach the summit of Mount Everest, astronauts are decompressed to these values within minutes to hours, thus making supplemental oxygen essential to prevent any of the altitude illnesses described earlier in this chapter. However, oxygen represents a major fire hazard to any spacecraft and no manned space programs currently use spacecraft with elevated oxygen levels for this reason. Therefore, some form of decompression procedure currently remains essential prior to any EVA. Although this may not be much of a concern to routine commercial passenger travel into space (passengers will not need to routinely exit their spacecraft), how best to manage these conflicting issues on longer missions to the moon or Mars remains unclear.
Microgravity
The human body has carefully evolved over millions of years to perform optimally in an environment with gravity. In fact, the effects of gravity on our environment are so ubiquitous that it is challenging to contemplate just how significant a role gravity plays on human health and performance. Without gravity, just attempting to function and interact appropriately with our surrounding environment becomes immensely difficult, and simple phenomena that we take for granted on earth no longer occur. Without gravity, there is also no convection, making temperature control difficult. As an example, burning flames are spherical and blue rather than yellow and pointy; there is no downward soot deposition or upward heat transfer and the flame burns as soon as it reaches any oxygen and in all directions. Without gravity, there is no effect of buoyancy—bubbles do not rise to the surface but remain suspended in fluids ( Fig. 74.3 ). In other words, to remove any bubbles from fluid in a syringe requires the application of an external accelerative force. Weightlessness has an impact on almost every organ system in the body, posing a number of both acute and chronic health risks to astronauts. A future mission to Mars raises further challenges as astronauts will need to be able transition from weightlessness to functioning in a gravitational environment again within minutes after landing.

Cardiovascular Physiology During Space Flight
The cardiovascular system is particularly susceptible to the effects of microgravity. Shortly after takeoff, an acute “fluid shift” quickly occurs as fluid usually held in the venous (high capacitance) side of the circulation starts to rapidly redistribute throughout other tissue beds ( Fig. 74.4 ). This effect is most marked in the upper body with up to 2 L of the blood normally pooled in the venous system in the legs by gravity is released upward into the central circulatory system within minutes of launching. Astronauts quickly develop facial edema and a so-called “chicken leg” syndrome as a result of this shift.

Different studies suggest that cardiac output increases between 18% and 30% acutely in response to weightlessness and that this increase persists for at least 8 days. This increase is driven predominantly by increases in stroke volume as overall HR appears to remain relatively unchanged. Systemic vascular resistance (SVR) also decreases by 14% to 35%, leaving systolic BP relatively unchanged, although some studies suggest that resting diastolic and mean arterial pressures might both decrease slightly in response to microgravity but still increase as normal in response to exercise. These decreases may turn out to be even more pronounced, however, on longer-duration space missions. Paradoxically, central venous pressure (CVP) acutely decreases after takeoff, possibly as a result of increased atrial distension. One study of space shuttle astronauts suggested that baroreflex sensitivity at rest might actually increase briefly initially before returning to baseline levels a few days later, but the baroreflex response seems to be chronically reduced after longer (e.g., 9 months) duration missions.
As time in space increases, adaptation to microgravity slowly decreases SV (and cardiac output) again and allows ejection fraction to increase. This is presumed to be driven by anatomical changes to cardiac tissue, as left ventricular mass and mean sphericity index may decrease by 8.0% and 9.6%, respectively, together with a drop in total body fluid.
Plasma volume decreases of up to 15% have been reported in both bedrest and spaceflight studies, accompanied by similar decreases in total red cell mass. Whereas plasma volume decreases within 24 hours, red cell changes take a week to become apparent, suggesting different mechanisms behind each change, but the exact cause of the decrease in red blood cells (RBCs) remains unclear. Elevated ferritin levels have been measured after space shuttle missions, but decreased reticulocyte counts suggest this process is because of reduced RBC production and not increased destruction/consumption. But this could also be a result of changes in tissue oxygen levels, particularly in the kidney, as a result of fluid shifting from the lower limbs toward the central (and renal) tissue beds.
Although these fluid changes reverse within 2 to 3 weeks after returning to Earth, initially hypovolemia and orthostatic intolerance still cause astronauts significant problems when they arrive back on Earth. Current protocols require astronauts to “fluid load” with salt water approximately 1 hour before landing, which significantly reduces these risks.
Microgravity itself does not appear to affect cardiac electrophysiology directly. However, a large number of arrhythmias have been reported during spaceflight and particularly during EVAs, possibly due to the many fluid shifts described above and the changes in catecholamine levels and adrenergic receptor sensitivity. Every one of the nine Skylab astronauts suffered some form of rhythm disturbance during their mission, NASA recorded multiple arrhythmias during its many space shuttle missions, and in 1987 one of the crewmembers on board the Russian MIR space station recorded a 14-beat run of ventricular tachycardia (VT).
Pulmonary Physiology During Space Flight
Human lungs are normally exquisitely sensitive to gravity, which induces relevant gradients to both ventilation distribution and pulmonary blood flow. Consequently, it is not surprising that pulmonary function alters during spaceflight away from the Earth’s normal gravity environment. However, adaptive changes remain remarkably short lived and, unusually, no pulmonary recovery or rehabilitation is commonly necessary when astronauts do return to Earth. Whether this would also remain true after future space missions with durations longer than 6 months currently remains unproven.
More specifically, respiratory rate increases by around 9% in microgravity but tidal volume decreases by up to 15%, meaning that minute alveolar ventilation remains essentially unchanged overall. Other respiratory measurements don’t alter significantly either with space flight. Measurements suggest that although vital capacity, forced vital capacity, and FEV1 may all decrease marginally (by around 2%-5%) within 24 hours of entering microgravity ( Fig. 74.5 ), by the fourth day of spaceflight all have returned to pre-flight values. Residual volume decreases by around 18% in space (approximately 320 mL) and consequently functional residual capacity also reduces by around 15% (approximately 500 mL) in microgravity, possibly because of cranial movement of the diaphragm, more uniform alveolar expansion, and increased intrathoracic blood flow. However, although ventilation and blood flow are more uniform throughout the lung in microgravity, a small and clinically insignificant degree of ventilation perfusion mismatch still remains even in space.

Image courtesy ESA.)
From European Space Agency. http://m.esa.int/spaceinimages/Images/2016/02/Taking_Tim_s_breath_away . Published 2016.
On average, ISS astronauts’ exercise capacity (as measured by <SPAN role=presentation tabIndex=0 id=MathJax-Element-3-Frame class=MathJax style="POSITION: relative" data-mathml='V˙O2′>V˙O2V˙O2
V ˙ O 2
peak) decreases in the initial stages of their space flight (by 17%) but then continues to gradually normalize again throughout the remainder of their mission. Although some astronauts were able to reach their pre-flight value, others were not, suggesting considerable interindividual variability in exercise-responses to spaceflight. The fact that aerobic capacity increasingly recovers as flight durations lengthen suggests this is a successful response to exercise countermeasures employed during and throughout each ISS mission. All astronauts undergo an individually tailored daily exercise regime whilst in space to counter any aerobic deconditioning, but this countermeasure program has continued to evolve over the years as both knowledge and the equipment available for use on the ISS has changed and improved. While the relative contribution of both treadmill running and cycle ergometry has steadily decreased over the last 10 years of ISS missions to just 30 minutes of each on average per day, the number of resistance exercises in these programs has gradually increased to around an hour a day. HR only increases marginally on return to Earth after long duration space flights, suggesting that these programs can successfully maintain astronauts’ cardiovascular fitness to enable them to meet the demands of daily mission activities. Another one of the main aims of this rigorous exercise regime is to counter the significant muscle atrophy and bone demineralization that occurs under conditions of microgravity ( Fig. 74.6 ).

Musculoskeletal Physiology During Space Flight
Marked bone demineralization starts immediately after entering space and leaving Earth’s gravity. Urinary and fecal calcium excretion increase by 60% to 70% in the first day of space flight, and continues throughout the mission’s duration along with notable increases in urinary levels of bone resorption markers and decreases in blood levels of both parathyroid hormone and 1,25 dihydroxy vitamin D. On Earth, the average loss of bone density as a result of aging is approximately 1% per year, whereas in space, bone density decreases an average 1% per month and the rate can be double in the pelvic bones. Exercise countermeasure programs (particularly resistive exercise elements) can mitigate these effects and are improving each year, but they are not able to curtail these effects and the risks of fragility fractures and renal stones during space flight remain high among astronauts (and are major concerns for future Mars mission planners), as do long-term health risks such as osteoporosis.
Similarly, studies of astronauts and cosmonauts on both U.S. space shuttle missions and the Russian Mir missions of 16 and 28 weeks’ duration demonstrated significant decreases (5%-17%) in muscle volume in all muscle groups except the neck. Muscle groups normally required to maintain an upright posture are usually most affected (i.e., antigravity muscles). For example, muscle volume loss in ISS crewmembers was greatest in the calves (10%-16% reduction) and in the thighs (4%-7% reduction), whereas no change was seen in upper limb muscle volume. Vastus lateralis muscle biopsies taken from 8 astronauts on early space shuttle missions up to 11 days long confirmed that relative losses were greatest in type IIB and then type IIA (fast twitch) muscle fibers, with type I (slow twitch) fibers changing the least. Functional muscle impairment also occurs alongside these structural muscle changes in response to microgravity, with increased muscle fatiguability and decreased muscle-fiber strength also seen most extensively in antigravity muscle groups. It is notable that decreases in muscle function during space flight are out of proportion with the loss of muscle structure alone. Other factors, including neuronal muscle control, may also contribute. A study by Antonutto found that maximal explosive power decreased between 45% and 65% in spaceflights of 1 to 3 months’ duration, while muscle mass only decreased by 9% to 13% during the same period. Electromyographic cycle period and burst durations were decreased in Rhesus monkeys after simulated spaceflight, and significant amounts of dendritic remodeling have been noted in rats on board the ISS.
Central Nervous System and Psychological Challenges During Space Flight
Astronauts commonly experience neurologic changes soon after they arrive in space and also rapidly after their return to Earth, with many of these deficits persisting for long periods after their return. For example, most crewmembers have had some form of locomotion dysfunction after transiting into or out of microgravity, such as ataxia or postural instability. Postural instability may be explained by a number of factors: differential muscle wasting affects postural tone; unloading of the spine means astronauts can gain 2 to 3 inches in height within 10 days of arriving in space, causing stretching of spinal nerve roots and altering the usual length-tension relationship of different muscle compartments (including antigravity muscles most affected by wasting in microgravity); and changes to vestibular feedback loops (the cerebellum and the vestibular system are usually finely tuned to the Earth’s gravity) can also significantly impair normal postural reflexes.
The vestibular system, composed of the semicircular canals and otoliths that sense distant angular and linear forces, respectively, is constantly updating the brain about the head’s three-dimensional orientation and movement. However, this system has evolved and developed over thousands of years to be finely tuned to the Earth’s gravitational field and not a microgravity environment, making vestibular problems in space extremely common. Between 60% and 80% of all astronauts will experience “space motion sickness” within 48 hours of arriving in space, a condition characterized by symptoms of pallor, anorexia, nausea, sometimes vomiting, increased body warmth, and cold sweating. It is usually a short-lived phenomenon, with most cases either resolving spontaneously or with minimal pharmacologic treatment within 48 to 72 hours, but occasionally symptoms can persist longer. Rarely has this condition led to crew members being incapacitated for an entire space shuttle mission. It poses a real challenge to astronauts contemplating missions of long duration to either the moon or to Mars, where they may need to function normally in a new gravitational environment very shortly after landing in order to survive.
Terrestrial motion sickness commonly results from discrepancies between visual and vestibular perceptions of motion. Similarly, lack of any vestibular sense of “up or down” in the unique, weightless environment of space may also contribute to the development of space motion sickness. Microgravity affects other sensory systems too. American and Russian astronauts have reported that foods taste and smell different in space with requests for spices to enhance many foods tasting more bland, as well as having less of a desire for coffee and sweets. Similarly, astronauts are constantly affected by noise during spaceflight with life support systems operating constantly. While ear plugs can block out the noise (typically at levels of 70 to 100 dBA running constantly in very close proximity, 24 hours a day), they do not attenuate the vibrations caused by these machines. However, the headward fluid shifts associated with microgravity may also impair middle ear function and may attenuate the sense of vibration to some degree. Microgravity also impairs proprioceptive sense of limb position and modifies tactile sensitivity with a significant degree of interindividual variability.
The visual system is particularly affected by spaceflight. Anatomical and functional changes, including disc edema, cotton wool spots, choroidal folds, nerve fiber layer thickening, globe flattening, and consequent hyperopic shifts in vision have all been reported in seven astronauts examined after returning from long-duration spaceflights on the ISS. In one of these astronauts, who had initially only experienced relatively mild changes after his 6-month flight (unilateral choroidal folds and a single cotton wool spot in the right eye), these changes continued to progress during a second 6-month mission and he developed disc edema and more widespread choroidal folds in the same eye. Soon after returning from this second mission, globe flattening and moderate increases in optic nerve sheath diameters were noted bilaterally. Twenty-one months later, widespread choroidal folds remained in the right eye and spontaneous venous pulsations were also absent. Choroidal folds in astronauts were thought to be caused by increased ICP during spaceflight (possibly secondary to higher rates of resistive exercise, higher atmospheric levels of CO 2 on board the spacecraft, or the cephalad fluid shifts associated with spaceflight), which are transmitted to the eye through increased levels of CSF transiting along the optic nerve sheath and putting more posterior pressure on both globes. In fact, this is “visual impairment intracranial pressure syndrome” as seen in spaceflight and it has similarities to some effects experienced by people visiting high-altitude regions. However, the long-term asymmetry of these findings in this particular subject suggests that ICPs may not be elevated over prolonged periods in space. Instead, visual impairment during spaceflight may be caused by localized changes to CSF flow within the intraorbital portion of the subarachnoid space—in effect causing an optic nerve sheath “compartment syndrome.”
Unsurprisingly, the effects of these changes on vision during spaceflight are widespread, and particularly during longer duration missions. Almost 30% of astronauts reported deteriorations in both near and distant visual acuity during short spaceflight missions, with this number increasing to 60% on long-duration missions. Older astronauts are more likely to notice changes as lens accommodation declines with age, and astronauts over the age of 40 are now routinely prescribed “Space Anticipation Glasses” to compensate for their new farsightedness in space. Anecdotal changes in visual fields and changes in intraocular pressure were also reported during the early years of the American space program and substantial amounts of evidence now show that transitioning to or from microgravity also impairs ocular movements and reflexes too.
In addition to coping with changes to their perception, astronauts also experience numerous psychologic challenges before, during, and after their mission. Astronaut selection is extremely competitive and the intensive international training schedule for crewmembers preparing for launch puts intense stresses on all members of the family unit even before the astronaut leaves Earth. Long periods of isolation have been shown to increase levels of stress as measured by activation of the hypothalamic-pituitary-adrenal axis (resulting in increased levels of cortisol production) and a degree of sleep impairment. The increased levels of insomnia astronauts experience is likely multifactorial and not simply explained by isolation alone. For example, low-earth orbit is associated with circadian desynchrony, increased levels of noise, hypoxia, hypercarbia, and extremes of temperatures. However, identifying how best to manage the psychologic stress caused by long-term isolation is critical to the success of any future long-term space missions. International space agencies now run a number of ground-based spaceflight research analog programs specifically to research this (and other) challenges to long-duration spaceflight. For example, the European Space Agency’s ground-based research analog program is based at the Concordia station situated high on the Antarctic plateau, which is completely inaccessible for half of the year, completely isolating the dozen crewmembers who “winter-over” there annually ( Fig. 74.7 ). Reports from the Mars 500 project, the first high-fidelity simulated mission to Mars isolating a multinational crew of 6 in a 550 m 3 chamber for 520 days, recently suggested that appropriate selection of crewmembers is key. Substantial interindividual differences were seen in behavioral responses: two crewmembers with the highest ratings of stress and exhaustion accounted for more than 85% of all of the perceived conflicts.
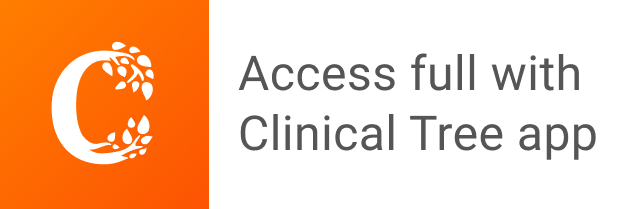