
Endothelial Function and Regulation of Vascular Tone
Endothelial synthesis and release of vasoactive mediators are important elements in the regulation of vascular tone. Substances are released by the endothelium in response to both mechanical and humoral stimuli and generally have an immediate effect upon the adjacent vascular smooth muscle tone. However, there may also be endothelium-induced long-term effects from vascular remodeling and smooth muscle hypertrophy. Under physiologic conditions, local vascular pressure and flow are the primary stimuli for endothelial vasoactive substance release. Nitric oxide and prostacyclin are powerful vasodilators released by endothelial cells and both also inhibit platelet aggregation and thrombosis. Continuous nitric oxide production maintains vascular tone in a normally low state. This minute-to-minute regulation of local vascular tone is controlled by type 3 constitutive nitric oxide synthase (cNOS). cNOS is a rapidly responding endothelial enzyme that catalyses the local conversion of L-arginine into small quantities of nitric oxide in response to endothelial shear stress during normal pulsatile flow. On the other hand, type 2 inducible NOS (iNOS) is a relatively slow-responding enzyme that catalyses the production of large amounts of nitric oxide in response to inflammatory cytokines. The widespread generation of large amounts of nitric oxide via iNOS is responsible for the low systemic vascular resistance and hypotension encountered in septic shock.3 Endothelin-1 (ET-1) is a potent vasoactive compound released by the endothelium. Its predominant effect is vasoconstriction via smooth muscle ETA receptors. However, ET-1 can also cause vasodilation through its effect on endothelial ETB receptors. ET-1 stimulates smooth muscle proliferation and is an important factor in the development of vascular structural changes in systemic and pulmonary hypertension.
Components of the Systemic Circulation
The components of the systemic circulation are the arteries, arterioles, capillaries, venules, and veins.
Arteries
The function of the arteries is to transport blood under high pressure to tissues. Therefore, arteries have strong vascular walls and blood flows rapidly through their lumens.
Arterioles
Arterioles are the last small branches of the arterial system, having diameters of less than 200 µm. Arterioles have strong muscular walls, which are capable of dilating or contracting and thus controlling blood flow into the capillaries. Indeed, blood flow to each tissue is controlled almost entirely by resistance to flow in the arterioles. Metarterioles arise at right angles from arterioles and branch several times, forming 10 to 100 capillaries which in turn connect with venules.
Capillaries
Capillaries are the sites for transfer of oxygen and nutrients to tissues and receipt of metabolic byproducts.
Venules and Veins
Venules collect blood from capillaries for delivery to veins, which act as conduits for transmitting blood to the right atrium. Because the pressure in the venous system is low, venous walls are thin. Nevertheless, walls of veins are muscular, which allows these vessels to contract or expand and thus store varying amounts of blood, depending on physiologic needs. As a result, veins serve an important storage function as well as being conduits to return blood to the right atrium. A venous pump mechanism is important for propelling blood forward to the heart.
Physical Characteristics of the Systemic Circulation
The systemic circulation contains about 80% of the blood volume, with the remainder present in the pulmonary circulation and heart (Fig. 14-1).4 Of the blood volume in the systemic circulation, about 64% is in veins and 7% is in the cardiac chambers. The heart ejects blood intermittently into the aorta such that blood pressure in the aorta fluctuates between a systolic level of about 120 mm Hg and a diastolic level of about 80 mm Hg (Table 14-3) (Fig. 14-2).4



One of the primary responsibilities of the anesthesiologist is to maintain organ perfusion with oxygenated blood. Our standard physiologic monitors (heart rate, blood pressure, pulse oximetry, capnography) all serve as surrogate markers of organ perfusion and oxygenation. Currently, our standard monitoring techniques do not allow us to directly monitor the level of perfusion in specific organs or tissues. However, various techniques for monitoring end-organ perfusion are being explored and will likely be increasingly used in the years to come.
Progressive Declines in Systemic Blood Pressure
As blood flows through the systemic circulation, perfusion pressure decreases progressively to nearly 0 mm Hg by the time blood reaches the right atrium (see Fig. 14-2).4 The decrease in systemic blood pressure in each portion of the systemic circulation is directly proportional to the resistance to flow in the vessels. Resistance to blood flow in the aorta is minimal, and mean arterial pressure decreases only 3 to 5 mm Hg as blood travels into arteries as small as 3 mm in diameter. Resistance to blood flow begins to increase rapidly in small arteries, causing the mean arterial pressure to decrease to about 85 mm Hg at the beginning of the arterioles. It is in the arterioles that resistance to blood flow is the highest, accounting for about 50% of the resistance in the entire systemic circulation. As a result, systemic blood pressure decreases to about 30 mm Hg at the point where blood enters the capillaries. At the venous end of the capillaries, the intravascular pressure has decreased to about 10 mm Hg. The decrease in systemic blood pressure from 10 mm Hg to nearly 0 mm Hg as blood traverses veins indicates that these vessels impart far more resistance to blood flow than would be expected for vessels of their large sizes. This resistance to blood flow is caused by compression of the veins by external forces that keep many of them, especially the vena cava, partially collapsed.
Pulse Pressure in Arteries
Pulse pressure reflects the intermittent ejection of blood into the aorta by the heart (see Table 14-3). The difference between systolic and diastolic blood pressure is the pulse pressure. A typical systemic blood pressure curve recorded from a large artery is characterized by a rapid increase in pressure during ventricular systole followed by a maintained high level of blood pressure for 0.2 to 0.3 second (Fig. 14-3). This plateau is followed by the dicrotic notch (incisura) at the end of systole and a subsequent, more gradual decrease of pressure back to the diastolic level. The dicrotic notch reflects a decrease in the intraventricular pressure and a backflow of blood in the aorta, which causes the aortic valve to close.

Factors that Alter Pulse Pressure
The principal factors that alter pulse pressure in the arteries are the left ventricular stroke volume, velocity of blood flow, and compliance of the arterial tree. The larger the stroke volume, the greater the volume of blood that must be accommodated in the arterial vessels with each contraction resulting in an increased pulse pressure. Pulse pressure also increases when capacitance increases for outflow. When systemic vascular resistance decreases, flow of blood from arteries to veins is accelerated. Pulse pressure is also increased in the presence of patent ductus arteriosus and aortic regurgitation, reflecting rapid runoff of blood into the pulmonary circulation or left ventricle, respectively. In this regard, attempts have been made to predict systemic vascular resistance by the position of the dicrotic notch relative to the diastolic pressure. A controlled study, however, failed to confirm a correlation between the position of the dicrotic notch and the calculated systemic vascular resistance (Fig. 14-4).5 An increase in heart rate while the cardiac output remains constant causes the stroke volume and pulse pressure to decrease. Pulse pressure is inversely proportional to the compliance (distensibility) of the arterial system. For example, with aging, the distensibility of the arterial walls often decreases (elastic and muscular tissues are replaced by fibrous tissue) and pulse pressure increases.

Transmission of the Pulse Pressure
There is often enhancement of the pulse pressure as the pressure wave is transmitted peripherally (Fig. 14-5).4 Part of this augmentation results from the progressive decrease in compliance of the more distal portions of the large arteries. Second, pressure waves are reflected to some extent by the peripheral arteries. Specifically, when a pulsatile pressure wave enters the peripheral arteries and distends them, the pressure on these peripheral arteries causes the pulse wave to begin traveling backward. If the returning pulse wave strikes an oncoming wave, the two summate, causing a much higher pressure than would otherwise occur.

Augmentation of the peripheral pulse pressure must be identified whenever systemic blood pressure measurements are made in peripheral arteries. For example, systolic pressure in the radial artery is sometimes as much as 20% to 30% higher than that pressure present in the central aorta, and diastolic pressure is often decreased as much as 10% to 15%. Mean arterial pressures are similar regardless of the site of blood pressure measurement in a peripheral artery.
Pulse pressure becomes progressively less as blood passes through small arteries and arterioles until it becomes almost absent in capillaries (see Fig. 14-2).4 This reflects the extreme distensibility of small vessels such that the small amount of blood that is caused to flow during a pulsatile pressure wave produces progressively less pressure increase in the more distal vessels. Furthermore, resistance to blood flow in these small vessels is such that flow of blood and, consequently, the transmission of pressure are greatly impeded.
Systemic Blood Pressure Measurement during and after Cardiopulmonary Bypass
Reversal of the usual relationship between aortic and radial artery blood pressures can occur during the late period of hypothermic cardiopulmonary bypass and in the early period after termination of cardiopulmonary bypass (Fig. 14-6).6,7 One mechanism proposed for this unpredictable and transient disparity (usually persists for 10 to 60 minutes after discontinuation of cardiopulmonary bypass) is a high blood flow in the forearm and hand after rewarming on cardiopulmonary bypass, causing an increased pressure drop along the normal resistance pathway provided by the arteries leading to the radial site. Conversely, others describe the appearance of this gradient with initiation of cardiopulmonary bypass, suggesting that the etiology is associated with events such as cross-clamping of the aorta occurring during initiation of cardiopulmonary bypass rather than rewarming or discontinuing cardiopulmonary bypass (Fig. 14-7).8,9 Failure to recognize this disparity may lead to an erroneous diagnosis and unnecessary treatment. Systemic blood pressure measured in the brachial artery is more accurate and reliable during the periods surrounding cardiopulmonary bypass, which are most likely to be associated with disparities between the aortic and radial artery blood pressures.10


Pulsus Paradoxus
Pulsus paradoxus is an exaggerated decrease in systolic blood pressure (>10 mm Hg) during inspiration in the presence of increased intrapericardial pressures (cardiac tamponade). During normal inspiration, the decrease in intrathoracic pressure increases the compliance of the pulmonary vasculature, which leads to a relative decrease in pulmonary venous return to the left ventricle. The resultant reduction in left ventricular preload decreases the stroke volume, which manifests as a mildly decreased systolic blood pressure during inspiration (<10 mm Hg). Cardiac tamponade causes an exaggeration of this change in blood pressure with respiration.
Pulsus Alternans
Pulsus alternans is alternating weak and strong cardiac contractions causing a similar alteration in the strength of the peripheral pulse. A variety of physiologic conditions are associated with pulsus alternans. Digitalis toxicity, varying degrees of atrioventricular heart block, and left ventricular dysfunction are commonly associated with pulsus alternans. In the setting of left ventricular dysfunction, pulsus alternans is caused by cyclic alterations in the contractile state of the heart. A reduced stroke volume increases end diastolic volume, which results in increased myocardial contraction and therefore increased ventricular emptying and blood pressure (per the Frank-Starling law). During the subsequent cardiac cycle, the lower filling pressures in the left ventricle result in a decreased stroke volume and therefore decreased ventricular emptying and blood pressure.
Electrical Alternans
Electrical alternans is a phenomenon where the amplitude of the QRS complex changes between heart beats. This electrocardiographic finding is seen in cardiac tamponade and pericardial effusion, where the heart essentially moves within the fluid-filled pericardial sac during contraction.
Pulse Deficit
In the presence of atrial fibrillation or ectopic ventricular beats, two beats of the heart may occur so close together that the ventricle does not fill adequately and the second cardiac contraction ejects an insufficient volume of blood to create a peripheral pulse. In this circumstance, a second heart beat is audible with a stethoscope applied on the chest directly over the heart, but a corresponding pulsation in the radial artery cannot be palpated. This phenomenon is called a pulse deficit.
Measurement of Blood Pressure by Auscultation
Measurement of blood pressure by auscultation uses the principle that blood flow in large arteries is laminar and not audible. If blood flow is arrested by an inflated cuff and the pressure in the cuff is released slowly, audible tapping sounds (Korotkoff’s sounds) can be heard when the pressure of the cuff decreases just below systolic blood pressure and blood starts flowing in the brachial artery. These tapping sounds occur because flow velocity through the constricted portion of the blood vessel is increased, resulting in turbulence and vibrations that are heard through the stethoscope. Diastolic blood pressure correlates with the onset of muffled auscultatory sounds. The auscultatory method for determining systolic and diastolic blood pressure usually gives values within 10% of those determined by direct measurement from the arteries.
The width of the blood pressure cuff will affect measurements; ideally, the width of the blood pressure cuff should be 20% to 50% greater than the diameter of the patient’s extremity. If the cuff is too narrow, the blood pressure will be overestimated. If the cuff is too large, the blood pressure may be underestimated.
Right Atrial Pressure
Right atrial pressure is regulated by a balance between venous return and the ability of the right ventricle to eject blood. Normal right atrial pressure is about 5 mm Hg, with a lower limit of about −5 mm Hg, which corresponds to the pressure in the pericardial and intrapleural spaces that surround the heart. Right atrial pressure approaches these low values when right ventricular contractility is increased or venous return to the heart is decreased by hemorrhage. Poor right ventricular contractility or any event that increases venous return (hypervolemia, venoconstriction) tends to increase right atrial pressure. Pressure in the right atrium is commonly designated the central venous pressure (CVP). Other factors that increase CVP include tension pneumothorax, heart failure, tamponade, pleural effusion, mechanical ventilation, positive end-expiratory pressure, Valsalva, pulmonary hypertension, and pulmonary embolism.
Jugular Venous Pressure
Jugular venous pressure or the pressure in the internal jugular vein mirrors the CVP. The normal jugular venous pressure reflects phasic changes in the right atrium and consists of three positive waves and three negative troughs (Fig. 14-8).11 Abnormalities of these venous waveforms may be useful in the diagnosis of various cardiac conditions (Table 14-4).11


Peripheral Venous Pressure
Large veins offer little resistance to blood flow when they are distended. Most large veins, however, are compressed at multiple extrathoracic sites. For example, pressure in the external jugular vein is often so low that atmospheric pressure on the outside of the neck causes it to collapse. Veins coursing through the abdomen are compressed by intraabdominal pressure, which may increase 15 to 20 mm Hg as a result of pregnancy or ascites. When this occurs, pressure in leg veins must increase above abdominal pressure. It is important to recognize that veins inside the thorax are not collapsed because of the distending effect of negative intrathoracic pressure.
Effect of Hydrostatic Pressure
Pressure in veins below the heart is increased and that in veins above the heart is decreased by the effect of gravity (Fig. 14-9).4 As a guideline, pressure changes 0.77 mm Hg for every centimeter the vessel is above or below the heart. For example, in a standing human, pressure in the veins of the feet is 90 mm Hg because of the distance from the heart to the feet. Conversely, veins above the heart tend to collapse, with the exception being veins inside the skull, where they are held open by surrounding bone. As a result, negative pressure can exist in the dural sinuses and air can be entrained immediately if these sinuses are entered during surgery.

Hydrostatic pressure affects peripheral pressure in arteries and capillaries as well as veins. For example, a standing human who has a systemic blood pressure of 100 mm Hg at the level of the heart has a blood pressure of about 190 mm Hg in the feet.
Venous Valves and the Pump Mechanism
Valves in veins are arranged so that the direction of blood flow can be only toward the heart. In a standing human, movement of the legs compresses skeletal muscles and veins so blood is directed toward the heart. This venous pump or skeletal muscle pump is usually sufficient to maintain venous pressure below 25 mm Hg in a walking human. If an individual stands immobile, the venous pump does not function. As a result, pressures in the veins and capillaries of the legs can increase rapidly, resulting in leakage of fluid from the intravascular space. Indeed, as much as 15% of the blood volume can be lost from the intravascular space in the first 15 minutes of quiet standing.
Varicose Veins
Valves of the venous system can be destroyed when the veins are chronically distended by increased venous pressure as occurs during pregnancy or in an individual who stands most of the day. The end result is varicose veins characterized by bulbous protrusions of the veins beneath the skin of the legs. Venous and capillary pressures remain increased because of the incompetent venous pump, and this causes constant edema in the legs of these individuals. Edema interferes with diffusion of nutrients from the capillaries to tissues, so there is often skeletal muscle discomfort and the skin may ulcerate.
Reference Level for Measuring Venous Pressure
Hydrostatic pressure does not alter venous or arterial pressures that are measured at the level of the tricuspid valve. As a result, the reference point for pressure measurement is considered to be the level of the tricuspid valve. An appropriate external reference point for the level of the tricuspid valve in a supine individual is 5 cm posterior to the sternum at the level of the 4th intercostal space. A precise hydrostatic point to which pressures are referenced is essential for accurate interpretation of venous pressure measurements. For example, each centimeter below the hydrostatic point adds 0.77 mm Hg to the measured pressure, whereas 0.77 mm Hg is subtracted for each centimeter above this point. The potential error introduced by measuring pressures above or below the tricuspid valve is greatest with venous pressures that are normally low. For example, an error introduced by 5 cm of hydrostatic pressure has a much greater influence on the clinical interpretation of CVP than arterial pressure.
The reason for lack of hydrostatic effects at the tricuspid valve is the ability of the right ventricle to act as a regulator of pressure at this site. For example, if the pressure at the tricuspid valve increases, the right ventricle fills to a greater extent, thereby decreasing the pressure at the tricuspid valve toward normal. Conversely, if the pressure decreases at the tricuspid valve, the right ventricle does not fill optimally and blood pools in the veins until pressure at the tricuspid valve again increases to a normal value.
Measurement of right atrial pressure is accomplished by using a transducer or a fluid-filled manometer referenced to the level of the tricuspid valve. A venous pressure measurement in mm Hg can be converted to cm H2O by multiplying the pressure by 1.36, which adjusts for the density of mercury relative to water (10 mm Hg equals 13.6 cm H2O). Conversely, dividing the CVP measurement in cm H2O by 1.36 converts this value to an equivalent pressure in mm Hg.
Blood Viscosity
Blood is a viscous fluid composed of cells and plasma. More than 99% of the cells in plasma are erythrocytes. As a result, leukocytes exert a minimal influence on the physical characteristics of blood. The percentage of blood comprising erythrocytes is the hematocrit, which to a large extent determines the viscosity of blood (Fig. 14-10).4 When the hematocrit increases to 60% to 70%, viscosity of blood is increased about 10-fold compared with water, and flow through blood vessels is greatly decreased. Plasma protein concentrations influence blood viscosity only minimally.

Viscosity exerts fewer effects on blood flow in capillaries than in larger vessels. This most likely reflects alignment of erythrocytes as they pass through small blood vessels rather than the random arrangement characteristic of flow through larger vessels. This alignment of erythrocytes, which greatly decreases the viscous resistance that occurs normally between cells, is largely offset by a decreased velocity of flow that greatly increases viscosity. The net effect may be that viscous effects in small blood vessels are similar to those that occur in large blood vessels.
Plasma is considered extracellular fluid that is identical to interstitial fluid except for the greater concentrations of proteins (albumin, globulin, fibrinogen) in plasma. These greater concentrations reflect the inability of plasma proteins to pass easily through capillaries into the interstitial spaces. The presence of albumin creates colloid osmotic pressure, which prevents fluid from leaving the capillaries.
Determinants of Tissue Blood Flow
Tissue blood flow is directly proportional to the pressure difference between two points (not absolute pressure) and inversely proportional to resistance to flow through the vessel. This relationship between flow, pressure, and resistance can be expressed mathematically as a variant of Ohm’s law, in which blood flow (amperes) is directly proportional to the pressure drop across two points (voltage) and inversely proportional to resistance (Fig. 14-11). Rearrangement of this formula emphasizes that pressure is directly proportional to flow times resistance. Likewise, resistance is directly proportional to pressure and inversely proportional to flow. Furthermore, resistance is directly proportional to viscosity of blood and the length of the vessel and inversely proportional to the fourth power of the radius of the vessel (doubling the radius of the vessel or intravenous catheter size decreases resistance to flow 16-fold [Poiseuille’s law]).

It is important to understand that resistance to blood flow cannot be measured but rather is a calculated value based on measurement of driving pressures and the cardiac output. For example, systemic vascular resistance is calculated as the difference between mean arterial pressure and right atrial pressure divided by cardiac output. Pulmonary vascular resistance is calculated as the difference between mean pulmonary artery pressure and left atrial pressure divided by the cardiac output. Resistance is expressed in dynes/s/cm−5 and is calculated by multiplying the equation for either systemic vascular resistance or pulmonary vascular resistance just described by a conversion factor of 80. Conductance is the reciprocal of resistance and is a measure of the amount of blood flow that can pass through a blood vessel in a given time for a given pressure gradient.
Vascular Distensibility
Blood vessels are distensible such that increases in systemic blood pressure cause the vascular diameter to increase, which in turn decreases resistance to blood flow. Conversely, decreases in intravascular pressure increase the resistance to blood flow. The ability of blood vessels to distend as intravascular pressure increases varies greatly in different parts of the circulation. Anatomically, the walls of arteries are stronger than those of veins. As a result, veins are 6 to 10 times as distensible as arteries. Systemic blood pressure can eventually decrease to a level where intravascular pressure is no longer capable of keeping the vessel open. This pressure averages 20 mm Hg and is defined as the critical closing pressure. When the heart is abruptly stopped, the pressure in the entire circulatory system (mean circulatory pressure) equilibrates at about 7 mm Hg.
Vascular Compliance
Vascular compliance is defined as the increase in volume (capacitance) of a vessel produced by an increase in intravascular pressure. The compliance of the entire circulatory system is estimated to be 100 mL for each 1 mm Hg increase in intravascular pressure.4 The compliance of veins is much greater than that of arteries. For example, the volume of blood normally present in all veins is about 2,500 mL, whereas the arterial system contains only about 750 mL of blood when the mean arterial pressure is 100 mm Hg. Sympathetic nervous system activity can greatly alter the distribution of blood volume. Enhancement of sympathetic nervous outflow to the blood vessels, especially the veins, decreases the dimensions to the circulatory system, and the circulation continues to function almost normally even when as much as 25% of the total blood volume has been lost. Vasoconstriction or vasodilation refers to resistance changes in arterioles, whereas changes in the caliber of veins are described as venoconstriction or venodilation.
Control of Tissue Blood Flow
Control of blood flow to different tissues includes local mechanisms, autonomic nervous system responses, and release of hormones. Total tissue blood flow or cardiac output is about 5 L per minute, with large amounts being delivered to the heart, brain, liver, and kidneys (Table 14-5).4 In contrast, skeletal muscles represent 35% to 40% of body mass but receive only about 15% of the total cardiac output, reflecting the low metabolic rate of inactive skeletal muscles.

Local Control of Blood Flow
Local control of blood flow is most often based on the need for delivery of oxygen or other nutrients such as glucose or fatty acids to the tissues. The response to decreased oxygen delivery may reflect the local release of vasodilatory substances (adenosine, lactic acid, carbon dioxide, potassium ions), which results in increased tissue blood flow and oxygen delivery.
Autoregulation of Blood Flow
Autoregulation is a local mechanism that controls blood flow in which a specific tissue is able to maintain a relatively constant blood flow over a wide range of mean arterial pressures. When the mean arterial pressure increases, the associated increase in tissue blood flow causes the blood vessels to constrict, thereby limiting any increase in blood flow. Conversely, decreases in mean arterial pressure result in vasodilation, which maintains tissue blood flow. Autoregulatory responses to sudden changes in mean arterial pressure occur within 60 to 120 seconds. The ability of autoregulation to return local tissue blood flow to normal is incomplete.
Long-term Control of Blood Flow
Long-term regulatory mechanisms that return local tissue blood flow to normal involve a change in vascularity of tissues. For example, sustained increases in mean arterial pressure to specific tissues, as occurs above a coarctation of the aorta, is accompanied by a decrease in the size and number of blood vessels. Likewise, if metabolism in a tissue becomes chronically increased, vascularity increases, or, if metabolism is decreased, vascularity decreases. Indeed, inadequate delivery of oxygen to a tissue is the stimulus for the development of collateral vessels. Neonates exposed to increased concentrations of oxygen can manifest cessation of new vascular growth in the retina. Subsequent removal of the neonate from a high-oxygen environment causes an overgrowth of new vessels to offset the abrupt decrease in availability of oxygen. There may be so much overgrowth that the new vessels cause blindness (retrolental fibroplasia).
Autonomic Nervous System Control of Blood Flow
Autonomic nervous system control of blood flow is characterized by a rapid response time (within 1 second) and an ability to regulate blood flow to certain tissues at the expense of other tissues. The sympathetic nervous system is the most important component of the autonomic nervous system in the regulation of blood flow; sympathetic stimulation causes release of norepinephrine, which stimulates α-adrenergic receptors to produce vasoconstriction. Constriction of small arteries influences resistance to blood flow through tissues, whereas venoconstriction alters vascular capacitance and distribution of blood in the peripheral circulation. Sympathetic nervous system innervation is prominent in the kidneys and skin and minimal in the cerebral circulation.
Vasomotor Center
The vasomotor center, which is located in the pons and medulla, transmits sympathetic nervous system impulses through the spinal cord to all blood vessels. Evidence for a continuous, sustained state of partial vasoconstriction (vasomotor tone) is the abrupt decrease in systemic blood pressure that occurs when sympathetic nervous system innervation to the vasculature is abruptly interrupted, as by traumatic spinal cord transection or regional anesthesia. Activity of the vasomotor center can be influenced by impulses from a number of sites, including diffuse areas of the reticular activating system, hypothalamus, and cerebral cortex. Sympathetic nervous system impulses are transmitted to the adrenal medulla at the same time they are transmitted to the peripheral vasculature. These impulses stimulate the adrenal medulla to secrete epinephrine and norepinephrine into the circulation, where they act directly on adrenergic receptors in the walls of vascular smooth muscle.
The medial and lower portions of the vasomotor center do not participate in transmission of vasoconstrictor impulses but rather function as an inhibitor of sympathetic nervous system activity, which allows blood vessels to dilate. Conceptually, this portion of the vasomotor center is functioning as the parasympathetic nervous system.
Mass Reflex
The mass reflex is characterized by stimulation of all portions of the vasomotor center, resulting in generalized vasoconstriction and an increase in cardiac output in an attempt to maintain tissue blood flow. The alarm reaction resembles the mass reflex, but associated skeletal muscle vasodilation and psychic excitement are intended to prepare the individual to confront a life-threatening situation.
Syncope
Emotional fainting (vasovagal syncope) may reflect profound skeletal muscle vasodilation such that systemic blood pressure decreases abruptly and syncope occurs. Associated vagal stimulation results in bradycardia. This phenomenon may occur in patients who have an intense fear of needles, resulting in syncope during placement of an intravenous catheter.
Hormone Control of Blood Flow
Vasoconstrictor hormones that may influence local tissue blood flow include epinephrine, norepinephrine, angiotensin, and arginine vasopressin (formerly known as antidiuretic hormone). Bradykinin, serotonin, histamine, prostaglandins, and low circulating concentrations of epinephrine are vasodilating substances. Local chemical factors, such as accumulation of hydrogen ions, potassium ions, and carbon dioxide, relax vascular smooth muscle and cause vasodilation. Carbon dioxide also has an indirect vasoconstrictor effect because it stimulates the outflow of sympathetic nervous system impulses from the vasomotor center.
Regulation of Systemic Blood Pressure
Systemic blood pressure is maintained over a narrow range by reciprocal changes in cardiac output and systemic vascular resistance. The autonomic nervous system and baroreceptors play a key role in moment-to-moment regulation of systemic blood pressure. Long-term regulation of blood pressure depends on control of fluid balance by the kidneys, adrenal cortex, and central nervous system.
Systolic, diastolic, and mean arterial pressure tends to increase progressively with age. Because a greater portion of the cardiac cycle is nearer the diastolic blood pressure, it follows that mean arterial pressure is not the arithmetic average of the systolic and diastolic blood pressures. Mean arterial blood pressure is the most important determinant of tissue blood flow because it is the average, tending to drive blood through the systemic circulation.
Rapid-Acting Mechanisms for the Regulation of Systemic Blood Pressure
Rapid-acting mechanisms for regulation of systemic blood pressure involve nervous system responses as reflected by the baroreceptor reflexes, chemoreceptor reflexes, atrial reflexes, and central nervous system ischemic reflex. These reflex mechanisms respond almost immediately to changes in systemic blood pressure. Furthermore, within about 30 minutes, these nervous system reflex responses are further supplemented by activation of hormonal mechanisms and shift of fluid into the circulation to readjust the blood volume. These short-term mechanisms can return systemic blood pressure toward but never entirely back to normal. Indeed, the impact of many of the rapid-acting regulatory mechanisms, such as the baroreceptor reflexes, diminishes with time as these mechanisms adapt to the new level of systemic blood pressure.
Baroreceptor Reflexes
Baroreceptors are nerve endings in the walls of large arteries in the neck and thorax, especially in the internal carotid arteries just above the carotid bifurcation and in the arch of the aorta (Fig. 14-12).12 These nerve endings respond rapidly to changes in systemic blood pressure and are crucial for maintaining normal blood pressure when an individual changes from the supine to standing position. An increase in mean arterial pressure produces stretch of baroreceptor nerve endings, and increased numbers of nerve impulses are transmitted to the depressor portion of the vasomotor center, leading to a relative decrease in the central nervous system outflow of sympathetic nervous system (vasoconstrictive) impulses (Fig. 14-13).12 The net effects are vasodilation throughout the peripheral circulation, decreased heart rate, and decreased myocardial contractility, which all act to decrease systemic blood pressure back toward normal. Conversely, decreases in systemic blood pressure reflexively produce changes likely to increase blood pressure. Baroreceptors adapt in 1 to 3 days to sustained changes in systemic blood pressure, emphasizing that these reflexes are probably of no importance in long-term regulation of blood pressure. Volatile anesthetics, particularly halothane, inhibit the heart rate response portion of the baroreceptor reflex that occurs in response to changes in systemic blood pressure (see Chapter 4).

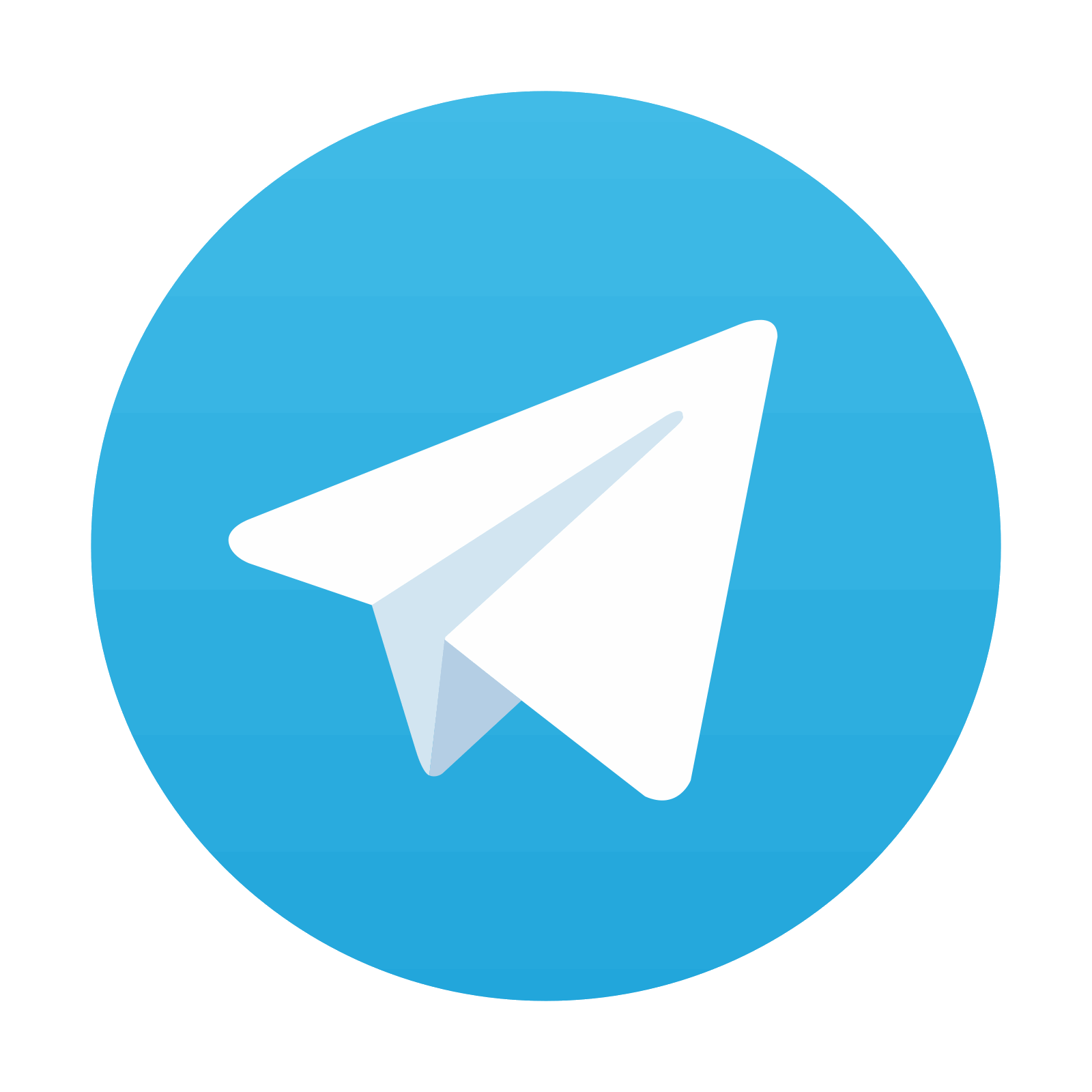
Stay updated, free articles. Join our Telegram channel
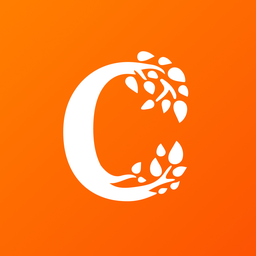
Full access? Get Clinical Tree
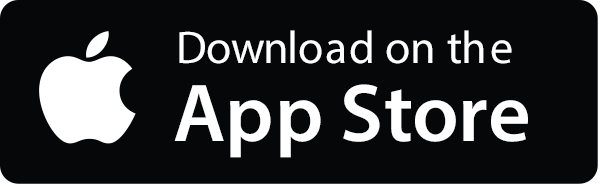
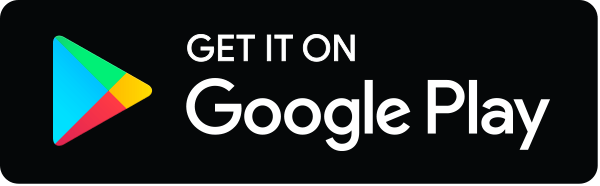