CHAPTER 27
Chronic Kidney Disease
Shaffeeulah Bacchus, PharmD, BCPS, FASN • Shukyu Tai Bacchus, PharmD, BS
The kidney has many important regulatory functions, such as regulation of body fluid volume, solute dilution and concentration, acid–base balance, and the metabolism and excretion of waste products. The kidney also secretes hormones that regulate red blood cell (RBC) production, blood pressure, and calcium homeostasis. Chronic kidney disease (CKD), regardless of cause, affects these vital processes, with changes seen throughout all organ systems. The kidneys exhibit remarkable adaptive abilities, and symptomatic changes usually do not become apparent until the glomerular filtration rate (GFR) declines to <25% of normal (Longo et al., 2011).
When dealing with CKD, proactive and early intervention is important. Although the progression of the disease is slow and presenting symptoms are usually nonemergent, the causative etiologies must be managed aggressively with specific pharmacology based on the underlying condition. Creatinine levels alone are not indicative of disease progression and may increase erratically or due to other causes not directly related to degree of kidney damage, such as changes in muscle mass or liver function. Patients with high risk for CKD should be monitored regularly for estimated glomerular filtration rate (eGFR), a laboratory value that is valuable for assessing the stage of kidney disease and therefore guiding early referral and/or intervention.
While monitoring the patient’s kidney function, the process that brought the patient to this stage must be investigated. The goal is to slow the progression toward end-stage kidney disease (ESRD) requiring dialysis and/or maintain the patient’s viability for a kidney transplant. To achieve this, it is important that the primary care provider become familiar with the anatomy and physiology of the kidney, as well as the most common causes of CKD.
EPIDEMIOLOGY
CKD affects approximately 16% of the adult population worldwide. The United States Renal Data System houses information on the incidence and prevalence of CKD and ESRD in the United States (U.S. Renal Data System [USRDS] 2012). The overall prevalence of CKD defined by an estimated GFR <60 mL/min/1.73 m2 or a urine albumin-to-creatinine ratio (ACR) of 30 mg/g or higher (eGFR <60 mL/min/1.73 m2) rose from 12.3% during 1988 to 1994 to 14% from 2005 to 2010, with the largest increase in patients with cardiovascular disease (CVD).
In 2010, the prevalent population of patients on hemodialysis was 383,992, those on peritoneal dialysis numbered 29,733, and those with a functioning kidney transplant numbered 179,361 bringing the total treated ESRD population to 593,086—a growth of 4% from 2009 that is the smallest increase in 30 years. The number of patients receiving hemodialysis is declining as those receiving peritoneal dialysis increases. This trend for home dialysis therapy may be a response to the Medicare bundled payment system that incentivizes home dialysis therapy. The incidence and prevalence of ESRD increase with increasing age, peaking in the eighth decade, with the mean age around 60 to 64 years. ESRD is more common in men than in women and is highest in the African American population.
The rate of ESRD for Blacks/African Americans and Native Americans in 2010 were 924 and 465 per million population, respectively, which continues to be higher than for Caucasian Americans. Thirteen percent of new ESRD patients in 2010 were Hispanic, and this rate has been stable over the past 2 years. Diabetes, hypertension, and primary glomerulonephritis are the three most common attributed causes of ESRD. The rate of new ESRD cases caused by diabetes has remained stable since 2000 at 152 per million population. In 2010, the rate of ESRD due to hypertension decreased by 2.2%, while that of glomerulonephritic ESRD fell to 22.7%.
Primary glomerulonephritis and cystic, hereditary, and congenital diseases are the most common causes of ESRD among patients younger than age 20 years. Diabetes predominates in persons aged 20 to 64 years, whereas hypertension is the largest attributed cause of ESRD in those older than 65 years. Primary glomerulonephritis and hypertension are more common in men than in women and diabetic ESRD is more common in women than in men. African Americans have a higher incidence of hypertensive ESRD than any other ethnic group. Asians have a higher incidence of primary glomerulonephritis, and Native Americans have the highest incidence of diabetic ESRD, with a relatively low incidence of hypertensive ESRD (USRDS, 2012).
The National Kidney Foundation published the 2002 Kidney Disease Outcomes Quality Initiative (KDOQI) CKD guidelines that defined CKD as either kidney damage (proteinuria, hematuria, or structural abnormalities of the kidney) with or without impaired eGFR, or impaired eGFR <60 mL/min/1.73 m2 with or without kidney damage for a period of 3 months or more.
The equation used to estimate GFR is the four-variable modification of diet in renal disease (MDRD) equation that was not validated in patients older than 70 years, nor in children, pregnant women, diabetics, Native Americans, or Hispanics. As a result, the chronic kidney disease (CKD) epidemiology equation (EPI) equation was proposed, utilizing pooled data from both CKD and non-CKD patients. This equation is also based on age, gender, and race and can therefore be adapted for automated eGFR reporting. Although the CKD EPI equation performed better than the MDRD equation in bias, precision was not significantly improved, but accuracy was greater for individuals with GFR >60 mL/min/1.73 m2 (Levey et al., 2009).
Table 27.1 is adapted from the National Kidney Foundation’s KDOQI Clinical Practice Guidelines for CKD: Evaluation, Classification, and Stratification (Kidney Disease: Improving Global Outcomes [KDIGO] CKD Work Group. KDIGO, 2012).
The CKD Prognosis Consortium, which was created by the Kidney Disease Improving Global Outcomes (KDIGO) Controversy Conference, examined studies that confirmed an independent and combined association for mortality in the presence of reduced eGFR, albuminuria measured by urine dipstick, and ACR (Levey et al., 2011; Matsushita et al., 2010). This led to a classification that combines eGFR and levels of proteinuria/albuminuria as a rationale for identifying patients with relatively normal ranges of eGFR but at high risk for CKD. Table 27.2 presents this classification.
NKF/KDOQI Classification of Stages of CKD |
STAGE | DESCRIPTION | GFR (mL/min/1.73 m2) |
1 | Kidney damage with none or increased GFR | >90 |
2 | Kidney damage with mild decreased GFR | 60–89 |
3 | Moderate decreased GFR | 30–59 |
4 | Severe decreased GFR | 15–29 |
5 | Kidney failure | <15 or dialysis |
CKD, chronic kidney disease; GFR, glomerular filtration rate; KDOQI, Kidney Disease Outcomes Quality Initiative; NKF, National Kidney Foundation.
Source: Adapted from the KDOQI Clinical Practice Guidelines for CKD: Evaluation, Classification, and Stratification (National Kidney Foundation, 2003).
The shading reflects the ranking of adjusted relative risk averaged over five outcomes (all-cause mortality, cardiovascular mortality, ESRD, acute kidney injury, and progressive CKD) for the 28 GFR and albuminuria categories. The categories with the mean rank numbers 1 to 8 are white, mean rank numbers 9 to 14 are light gray, mean rank numbers 15 to 21 are dark gray, and mean rank numbers 22 to 28 are black. The patterned black cells are extrapolated from studies, termed nephrotic, and have the highest level of albuminuria.
ANATOMY, PATHOPHYSIOLOGY, AND DIAGNOSIS
Anatomy
The kidneys are paired organs located on the posterior abdominal wall, outside the peritoneal cavity. They lie on either side of the vertebral column, with upper and lower poles extending from the twelfth thoracic to the third lumbar vertebrae. Each kidney is approximately 11 cm long, 5 to 6 cm wide, and 3 to 4 cm thick. The right kidney is displaced downward by the overlying liver and thus is lower than the left kidney. Each kidney is surrounded by a tightly adhering kidney capsule and embedded in a mass of fat. The capsule and fatty layer are covered with a double layer of kidney fascia, a fibrous tissue that attaches the kidney to the posterior abdominal wall. The medial indentation, called the hilus, serves as the entry and exit site for the kidney blood vessels, nerves, lymphatic vessels, and ureters. Figure 27.1 illustrates the anatomy of a kidney nephron (Carol, 1998).
The major components of the kidney are the kidney cortex and the kidney medulla. The kidney cortex contains all the glomeruli and portions of the tubules. The kidney medulla contains a series of wedges called the kidney pyramids. These pyramids are divided into outer and inner zones. The kidney columns extend from the kidney cortex down between the pyramids. The apex, which is the point of the kidney pyramid, extends down into the minor calyx—a cup-shaped cavity—and joins with other minor calyxes to form the major calyx. The major calyxes join to form the kidney pelvis, an extension of the upper end of the ureter.
Internal Structure
The nephron is the functional unit of the kidney (Figure 27.1). There are approximately 1.2 million nephrons per kidney. The nephron is a tubular structure made up of many subunits. The glomerulus is the tuft of capillaries that loop into a circular space called the Bowman’s capsule. The space within the capsule, known as Bowman’s space, descends toward the kidney medulla. Bowman’s capsule then merges into the proximal convoluted tubule. The proximal convoluted tubule is made up of two segments. The initial convoluted segment is the pars convoluta. The straight separate segment is the pars recta, which descends to the kidney medulla, forming the loop of Henle. As the loop of Henle emerges from the kidney medulla, it becomes the distal convoluted tubule. The distal tubule is formed by straight and convoluted segments and extends from the macula densa to the collecting ducts. These ducts are large tubules that descend down the kidney cortex and through the kidney pyramids of the inner and outer medulla into a minor calyx. The glomerulus is lined with epithelial cells. Mesangial cells lie between the capillaries of the glomeruli and hold them together, forming Bowman’s capsule.
There are two kinds of nephrons. First are the cortical nephrons, which extend only partially into the kidney medulla. They originate close to the surface of the kidney cortex. The juxtamedullary nephrons, the second type, extend deep into the medulla. They are important for concentration of urine. The juxtamedullary nephrons are located deep inside the kidney cortex, close to the kidney medulla. There are structural differences between the two types of nephrons. The cortical nephrons have a short loop, so they may not extend into the kidney medulla. The juxtamedullary nephrons have a loop that may extend the whole length of the medulla (40 mm); these represent approximately 12% of all nephrons.
The walls of the glomerular capillaries are comprised of three layers that act as a filtration membrane. The first is the inner capillary endothelium, which is composed of cells in continuous contact with the basement membrane. It is perforated by many small openings called fenestrae. The second layer is the middle basement membrane, a selectively permeable network of glycoproteins and mucopolysaccharides. The third layer is the epithelium. This layer has specialized cells called podocytes, footlike structures that radiate from the epithelium and adhere to the basement membrane. The pedicle of one podocyte interlocks with the pedicle of an adjacent podocyte, forming an elaborate network of intercellular clefts called filtration slits. The glomerular filtration membrane separates the blood of the glomerular capillaries from the fluid in Bowman’s space. The glomerular filtrate passes through the three layers of the glomerular membrane and forms the primary urine.
As the glomerular filtrate leaves Bowman’s capsule, it enters the proximal tubular lumen that consists of one layer of cells. This is the only surface inside the nephron that contains microvilli (a brush border). The microvilli create an expanded surface area inside the tubule, enhancing its reabsorptive function. The glomerular filtrate that is not reabsorbed in the proximal tubule then enters the loop of Henle. The loop is composed of two separate segments: a thin segment composed of thin squamous cells with no active transport, and a thick segment. This area is constructed of cuboidal cells, and actively transports several solutes out of the filtrate.
Toward the end of the loop of Henle, at its ascending loop, a transitional segment is formed. This is known as the macula densa. Here the distal tubule coming off the ascending loop passes between and contacts the afferent and efferent arterioles at their point of attachment to the macula densa and glomerulus. This linkage forms the juxtaglomerular apparatus. The juxtaglomerular apparatus has the important function of controlling kidney blood flow, glomerular filtration, and renin secretion. Specialized cells in the walls of afferent and efferent arterioles secrete the hormone renin that contributes to the control of arterial blood pressure.
Blood Vessels
The kidney arteries arise from the fifth branch of the abdominal aorta. As the kidney artery enters the kidney at the kidney hilus, it divides into anterior and posterior branches. These branches further divide into lobar arteries. The lobar arteries supply blood to the lower, middle, and upper thirds of the kidney. The interlobar arteries are further subdivisions of the lobar arteries that travel down between the kidney columns and between the kidney pyramids. At the corticomedullary junction, the interlobar arteries branch into the arcuate arteries. These vessels arch over the base of the kidney pyramids and run parallel to the surface of the kidney. They then branch into the interlobular arteries that extend through the kidney cortex toward the periphery and form the afferent arterioles. These arterioles subdivide into fistlike structures of four to eight glomerular capillaries. The blood supply proceeds through the glomerular capillaries and empties into the efferent arterioles, conveying blood into a second capillary bed, the peritubular capillaries. This is the only place in the body where an arteriole is positioned between two capillary beds. The positioning of the peritubular capillaries is important for glomerular filtration. The capillaries surround the convoluted portions of the proximal and distal tubules of the loop of Henle.
There are two types of peritubular capillaries. The first type supplies the cortical nephrons. These capillaries are similar to the capillaries of other tissues. The second are the capillaries that supply the juxtamedullary nephrons. These capillaries, called vasa recta, form loops and closely follow the loop of Henle. The peritubular capillaries of the vasa recta are the capillaries that influence the osmolar concentration of the medullary extracellular fluid that is important for the formation of concentrated urine. These capillaries are the only blood supply to the kidney medulla. All capillaries then drain into the venous system. The kidney veins follow the same path as the kidney arteries, only in the reverse direction.
PATHOPHYSIOLOGY
The kidney is a highly vascular organ, receiving approximately 20% to 25% of the cardiac output (1,000–1,200 mL blood/min). Approximately 20% of the plasma is filtered (120–140 mL/min) at the glomerulus and passes into Bowman’s capsule. The GFR is the filtration of plasma per unit of time. The GFR is directly related to the perfusion pressure in the glomerular capillaries. The rest of the plasma (about 80%) flows through the efferent arterioles to the peritubular capillaries. The ratio of glomerular filtration to kidney plasma flow per minute is called the filtration fraction. All but 1 to 2 mL of the glomerular filtrate is normally reabsorbed and returned to the circulation by the peritubular capillaries.
A common feature of CKD is the diminishing number of functioning or intact nephrons that operate normally. A theory known as the intact nephron hypothesis proposes that the loss of nephron mass with progressive kidney damage causes the remaining nephrons to sustain normal kidney function. These nephrons are capable of a compensatory expansion in their state of reabsorption and secretion. They also can maintain a constant rate of excretion in the presence of a declining GFR. The increased workload is achieved primarily by hypertrophy of the remaining nephrons. The intact nephron hypothesis explains the adaptive changes in solute and water regulation that occur with advancing kidney failure. As nephrons adapt during this compensatory phase, there is an increase in glomerular hyperperfusion and hypertrophy resulting in glomerulosclerosis, the underlying cause of many kidney diseases.
Another theory proposes an adaptive response by the nephrons. This adaptive response depends on the location of kidney damage. For example, with tubular interstitial disease, damage occurring primarily in the tubular or medullary parts of the nephron produces problems such as renal tubular acidosis, salt-wasting, and difficulty with dilution, and concentration of the urine. Conversely, when there is primarily vascular or glomerular damage, persistent hematuria and nephrotic syndrome are more prominent. This theory is useful for planning treatment in the early stages of kidney failure, when symptomatic differences in kidney diseases may be distinct.
Early management of these maladaptive compensatory mechanisms, such as with the use of angiotensin-converting enzyme inhibitors (ACEi) may reduce the progression of nephron loss.
Kidney Function
The function of the nephron is to form a filtrate of protein-free plasma. This process, known as ultrafiltration, occurs across the glomerular capillaries. The nephron then regulates the filtrate to help maintain body fluid volume and electrolyte composition within narrow limits. This fluid regulation happens through two processes. The first, tubular reabsorption, consists of movement of fluids and solutes from the proximal convoluted lumen into the peritubular capillary plasma. The second process, tubular secretion, is the active and passive transfer of substances from the plasma of the peritubular capillary into the lumen of the proximal convoluted tubule.
Glomerular Filtration
The total volume filtered by the kidney in 24 hours is approximately 180 liters (L). Changes in the GFR can occur with changes to the arterioles. For instance, constriction of the afferent arteriole will decrease the amount of blood entering the Bowman’s capsule, thereby decreasing the GFR. In contrast, constriction of the efferent arteriole will cause an increase in the pressure in the capillaries inside Bowman’s capsule, causing an increase in GFR. An endpoint to this is an increase in urinary excretion. By the time the filtrate travels to the end of the proximal tubule, 60% to 70% of the filtered sodium and water and 50% of the urea have been reabsorbed. In addition, 90% of the potassium, glucose, bicarbonate, calcium, phosphate, and uric acid have also been reabsorbed. All this is done by active transport. In the proximal tubule, active reabsorption of sodium is the primary function. Co-transported with the sodium are water, most electrolytes, and other organic substances.
At the loop of Henle, concentration or dilution of urine occurs, with additional concentration or dilution at the collecting ducts. When the process occurs at the loop of Henle, the strength of the concentrated filtrate is related to the length of the loop and the depth of penetration the loop has into the kidney medulla. The primary function of the loop is to establish a hyperosmotic state within the medullary interstitial fluid. Each portion of the loop of Henle is permeable to different aspects of the filtrate. The thin descending segment is highly permeable to water and moderately permeable to sodium, urea, and other solutes. The thin ascending segment is more permeable to sodium but almost impermeable to water. The thick ascending portions of the loop of Henle are highly permeable to sodium, potassium, and chloride and less so to urea and water. The convoluted portion of the distal tubule is poorly permeable to water but readily absorbs ions and contributes to the dilution of the tubular fluid. Finally, the straight segment of the distal tubule and the collecting duct are permeable to water and sodium. The absorption rate of the water is controlled by antidiuretic hormone (ADH). Sodium is readily absorbed by the distal tubule and collecting duct under the regulation of the hormone aldosterone.
GFR is directly related to kidney blood flow and regulated by intrinsic and extrinsic mechanisms:
1. Intrinsic autoregulatory mechanisms that keep the GFR stable over changing mean arterial pressures (80–180 mmHg) are myogenic or responsive to vascular changes due to fluctuations in kidney blood flow and pressure resulting in regulation of solutes and water filtration. In addition, tubuloglomerular feedback is triggered by changes in the concentration of sodium ions eliciting vascular responses in afferent arterioles to regulate GFR.
2. Extrinsic mechanisms are activation of the sympathetic autonomic nervous system and the renin–angiotensin–aldosterone system (RAAS) to increase systemic blood pressure and GFR. Sympathetic activation commonly due to physiologic stressors results in release of hormones such as epinephrine that causes vasoconstriction. Activation of the RAAS results in release of renin and may be caused by any of the following factors:
Decreased plasma sodium
Decreased plasma potassium
Decreased blood pressure in the afferent arterioles (this reduces the stretch of the juxtaglomerular cells)
Sympathetic stimulation of the beta-adrenergic receptors on the juxtaglomerular cells
The release of renin cleaves an alpha-globulin molecule (angiotensinogen) in the plasma to form angiotensin I. In the presence of angiotensin-converting enzyme (ACE), angiotensin I is converted to angiotensin II, which stimulates angiotensin receptors and the secretion of aldosterone by the kidney cortex. A potent vasopressor, aldosterone in turn inhibits renin release (a negative biofeedback mechanism).
Clinical Pearls
In response to increases or decreases in GFR, kidney tubules (primarily the proximal tubules) automatically adjust their rate of reabsorption of sodium and water to balance the changes in GFR.
Urine
Urea is an end product of protein metabolism and is the major constituent of urine, along with water. The glomerulus freely filters urea, and tubular reabsorption of urea depends on the urine flow rate. There is less reabsorption at higher flow rates. About 50% of urea is excreted in the urine; the other 50% is recycled within the kidney. The recycling of urea from the tubules and collecting ducts contributes to an osmotic gradient in the kidney medulla and is necessary for the concentration and dilution of urine. Because urea is an end product of protein metabolism, persons with protein deprivation will not be able to concentrate their urine maximally.
The final concentration of urine is controlled by ADH. ADH increases the permeability to water in the last segment of the distal tubule and along the entire length of the collecting ducts. Therefore, in the presence of ADH, water reabsorption is high. Water diffuses into the ascending limb of the vasa recta and returns to the systemic circulation. Excreted urine can have a high osmotic concentration (up to 1,400 mOsm). The volume is normally reduced to about 1% of what is filtered at the glomerulus.
ADH secretion is one cause of oliguria (urine excretion of <30 mL/hr or 400 mL/d). In the absence or inhibition of ADH, water diuresis takes place. The distal tubule and collecting ducts become impermeable to water. The water remains in the tubular lumen and is excreted as a diluted and large volume of urine. ADH has no effect on sodium reabsorption; sodium continues to be transported actively from the distal tubule and back into the kidney vasculature.
Acid–Base Balance
The normal diet produces 50 to 100 mEq of hydrogen per day. These ions are secreted in the kidney tubules and excreted in the urine, combined with phosphate and ammonia buffers. In the early stages of kidney disease, individual nephrons maintain normal pH by increasing the rate of acid excretion and bicarbonate absorption. Metabolic acidosis begins when the GFR falls below 30% to 40%, occurring primarily because of decreased ammonia synthesis and decreased bicarbonate reabsorption at each nephron. Phosphate buffers remain effective until very late in the process. When ESRD develops, serum bicarbonate levels stabilize at 15 to 20 mEq/L. This is partly because the excess hydrogen ions are buffered by anions in skeletal bone.
The metabolism of calcium and phosphate is mediated by parathyroid hormone (PTH) and vitamin D. Changes in the acid–base balance also influence the status of calcium and phosphate. The major disorders associated with CKD are reduced kidney phosphate excretion, decreased synthesis of activated vitamin D (1,25-OH2D3), and hypocalcemia. In the early stages of CKD, as the GFR falls, urinary excretion of phosphate falls and the plasma phosphate concentration rises. This results in phosphate binding with calcium to form calcium phosphate, which leads to hypocalcemia. The subsequent decrease in serum calcium triggers increased PTH secretion and this in turn stimulates the release of calcium from bone. This adaptive effect is a cause of secondary hyperparathyroidism.
With each incremental decrease in the GFR, the effectiveness of PTH in maintaining phosphate balance decreases. When the GFR is <25%, PTH is no longer effective in maintaining serum phosphate levels. The persistently decreased GFR and secondary hyperparathyroidism result in progressive hyperphosphatemia, hypocalcemia, and dissolution of skeletal bone. Hypocalcemia and bone disease are accelerated by the impaired synthesis of l,25-OH2D3. This decrease in activated vitamin D usually leads to reduced intestinal absorption of calcium. This impairs the effectiveness of calcium and phosphate reabsorption from the bone by PTH. The toxicity of uremia may also suppress vitamin D action in the intestines. The development of uremia can precipitate the development of uremic osteodystrophy, a bone disease caused by the loss of calcium from the bone as a result of increased serum phosphorus levels. This depletion can be treated with vitamin D supplementation. A negative calcium balance also occurs when the acidosis that is common in CKD is present.
Kidney Hormones
Vitamin D is a hormone that can be obtained from the diet as ergocalciferol (vitamin D2) or synthesized from cholesterol by the action of ultraviolet light in the skin to cholecalciferol (vitamin D3). These forms of vitamin D from the diet or skin are inactive and require two hydroxylations, one hepatic and one kidney, to establish the metabolically active form, 1,25-dihydroxycholecalciferol (OH2D3) or calcitriol. Calcitriol is necessary for the absorption of calcium and phosphate by the small intestine. A decreased calcium level (<10 mL/dL) stimulates the secretion of PTH that in turn stimulates the kidney hydroxylation step in the formation of calcitriol.
Serum phosphate fluctuation can also influence the kidney hydroxylation of vitamin D. As serum phosphate levels decrease, active l,25-OH2D3 formation increases; as serum phosphorus levels increase, active l,25-OH2D3 formation is inhibited. This results in compensatory phosphate absorption from the bone and intestine. The clinical significance of the role of the kidney in calcium and phosphate metabolism is evident in disease. Patients with CKD have deficiency of l,25-OH2D3 and manifest symptoms of disturbed calcium and phosphate balance.
Erythropoietin is a kidney hormone that stimulates the bone marrow to produce RBCs in response to perceived tissue hypoxia. The stimulus for increased erythropoietin release is a lowered oxygen delivery to the kidneys. As kidney function declines, the loss of intact nephrons results in a subsequent decrease in production of erythropoietin, leading to anemia of CKD.
Natriuretic hormone also is referred to as atrial natriuretic peptide (ANP). Though not a kidney hormone, ANP can affect the loss of sodium and water and the regulation of fluid balance. When the extracellular fluid volume is expanded, ANP secretion is increased and sodium reabsorption is depressed. This causes increased amounts of sodium and water to be excreted in the urine. ANP is released from cardiocyte granules located in the atria of the heart in response to the increased atrial stretch. However, as CKD progresses the renal effects of ANP in hypertensive or hypervolemic states may be blunted.
DIAGNOSTIC CRITERIA
Renal impairment refers to a decline in kidney function to about 25% of normal kidney filtration, or a eGFR of 25 to 30 mL/min. Serum chemistries show the mildly elevated levels of serum creatinine and blood urea nitrogen (BUN). A significant loss of kidney function (approximately 10%–25%) is termed kidney failure. When <10% of kidney function remains, this is termed ESRD. Kidney failure can be chronic, with progression to ESRD over a period of months to years.
Uremia is a syndrome of failure that includes elevated BUN and creatinine levels, accompanied by fatigue, anorexia, nausea, vomiting, pruritus, and neurologic changes. Electrolyte disorders and retention of toxic waste can also accompany this syndrome. Azotemia is a term sometimes used interchangeably with uremia. Both azotemia and uremia indicate an accumulation of nitrogenous waste products in the blood.
Although the urine of a patient with CKD may contain abnormal amounts of red and white blood cells and casts, the major end products of excretion are similar to those of normally functioning kidneys. There is no significant alteration to the urine until advanced stages of kidney disease, when there is a significant reduction of functioning nephrons.
Urinary excretion of potassium is primarily related to distal tubular secretion that is mediated by aldosterone and sodium–potassium ATPase. In kidney failure, the distal tubules and large intestine adapt to the effects of aldosterone and other factors to enhance the secretion of potassium. This provides effective regulation of potassium until the onset of oliguria. The symptoms of hyperkalemia are usually not evident in CKD until the GFR falls below 5 mL/min. When the GFR is greater and clinical symptoms are present, endogenous sources of increased serum potassium, such as trauma, infection, or hemolysis, should be investigated. Volume depletion, as well as the use of beta-blockers, ACE inhibitors, and potassium-sparing diuretics (e.g., spironolactone) may also precipitate an increase in the potassium level. The use of the antirejection medication cyclosporine can also elevate potassium levels. With the progression of the disease to ESRD, total serum potassium can increase to life-threatening levels and must be controlled by dialysis.
HISTORY AND PHYSICAL EXAMINATION
The history and physical examination involve a series of diagnostic tests, described in the following section.
DIAGNOSTIC STUDIES
Kidney clearance techniques determine how much of a substance can be cleared from the blood by the kidneys per a given unit of time. The application of this principle permits an indirect measure of GFR, tubular secretion, tubular reabsorption, and kidney blood flow.
GFR provides the best estimate of the amount of functioning kidney tissue. The loss of or damage to nephrons leads to a corresponding decrease in GFR. The GFR is calculated by multiplying the urinary creatinine value by the amount of urine produced for a given duration, usually 24 hours. This value is divided by the plasma creatinine value. Normal values are 130 ± 20 mL/min in males and 120 ± 15 mL/min in females.
Creatinine is a natural substance produced by the muscle; it is released into the blood at a relatively constant rate, and this is a commonly used clinical indicator. It is freely filtered at the glomerulus, but a small amount is released by the kidney tubules. Therefore, creatinine clearance may overestimate the GFR, but within tolerable limits. Creatinine clearance provides a fair measure of GFR, particularly when total daily urine is assessed. Creatinine clearance may be estimated by the Cockcroft and Gault equation: ([140 – age]/serum creatinine) x (ideal body weight/72). Inulin measurement is the gold standard for estimating GFR, as inulin is not naturally occurring in the body, is completely filtered at the glomerulus, and is neither secreted nor reabsorbed by the tubules. However, it requires an infusion and therefore is not practical in clinical practice. The MDRD equation (see the previous section, “Epidemiology”) allows for more variables, such as age, gender, and race; it is useful in understanding the stages of CKD and formulating an action plan for management, but remains less applicable to drug dosing (see “Medication Safety and Dosing” section).
The clearance of urea is similar to that of creatinine. Because urea is both filtered and reabsorbed, and the level varies with the state of hydration and diet, urea clearance is less than the GFR. If protein intake and metabolism are constant, plasma levels will increase as the GFR declines. Adaptation by the tubules does not modify urea levels because urea is excreted primarily by glomerular filtration.
A chronic decline in the GFR over weeks or months is reflected in the plasma creatinine concentration; normal values are 0.7 to 1.2 mg/dL. The plasma creatinine concentration is stable when the GFR is stable because creatinine has a constant rate of production as a product of muscle metabolism. The amount filtered is about equal to the amount excreted. When GFR decreases, the plasma creatinine level increases proportionately. Thus, the GFR and plasma creatinine are inversely related. If GFR decreases by 50%, the filtration and excretion of creatinine would be decreased by 50%, and creatinine would accumulate in plasma to twice the normal value. Therefore, an elevated plasma creatinine volume represents a decrease in the GFR.
This test is helpful for monitoring progressive changes in kidney function. It is most valuable for monitoring the progress of CKD rather than acute kidney injury because it takes 7 to 10 days for the plasma creatinine level to stabilize when the GFR declines. Plasma creatinine levels become elevated during trauma or breakdown of muscle tissue.
The concentration of BUN reflects glomerular filtration and the urine-collecting capacity of the kidney. Because urea is filtered at the glomerulus, BUN levels rise as the GFR decreases. Because urea is reabsorbed by the blood through the permeable tubules, BUN rises in states of dehydration. There is also a rise of BUN with acute and chronic kidney failure when passage of the glomerular filtrate through the tubules is slowed. Altered protein intake and protein catabolism can also alter BUN. Normal values of BUN in an adult are 10 to 20 mg/dL.
Urinalysis
During urinalysis, the color, turbidity, protein, pH, specific gravity, sediment, and supernatant are evaluated. Color is normally light yellow. When formed substances (crystals, blood cells, or casts) are in the urine, it appears turbid. Protein in the urine creates foam when shaken and bile pigment makes the urine foam yellow.
Urine pH ranges from 5 to 6.5, but it can vary from 4 to 8. Urine is more alkaline postprandially and then acidifies before the next meal. Because sleep is accompanied by intermittent hypoventilation, a person produces more acidic urine after awakening.
Specific gravity is an estimated measure of the solute concentration of the urine. This value is compared to the same volume of distilled water. Although specific gravity is not a true measure of the number or concentration of particles in the urine, it correlates well with osmolality. The normal values are 1.016 to 1.022.
Urine osmolality is primarily a function of ADH that controls water reabsorption in the collecting ducts. If the kidneys cannot concentrate a dilute urine with a given stimulus, the cause is usually a malfunction of the kidney tubules or inappropriate ADH secretion by the posterior pituitary gland. Hydration status also affects the urine specific gravity, so hydration status should be evaluated before making a diagnosis. This determination is helpful for differentiating oliguria caused by intrinsic kidney disease from hypovolemia as a result of dehydration.
Urinary sediment can represent cells, casts, crystals, and bacteria. Epithelial cells may be seen, as they are shed naturally throughout the urinary tract. Normal urine contains few or no RBCs. Hematuria is the term used when large numbers of RBCs are present in the urine. The sediment may be red when hematuria is present. An alkaline or hypotonic urine causes lysis of the RBCs, so the cells will not be seen. When RBCs are present, the urine will be positive for hemoglobin and the specific gravity will be elevated.
Casts are accumulations of cellular precipitates. They originate in the kidney tubules (from which they take their shape). They are cylindrical and have distinct borders. All casts arise primarily from the ascending limb of the distal tubule. RBC casts indicate bleeding into the tubule. White cell casts are associated with an inflammatory process. Epithelial cell casts indicate degeneration of the tubular lumen or necrosis of kidney tubules.
Crystals are composed of cystine, uric acid, calcium oxalate, or phosphate. They tend to form in a concentrated acidic or alkaline urine. They are generally not clinically significant. Diagnostically, they indicate an inflammation, infection, or metabolic disorder.
CONSIDERATIONS IN THE MANAGEMENT OF CKD
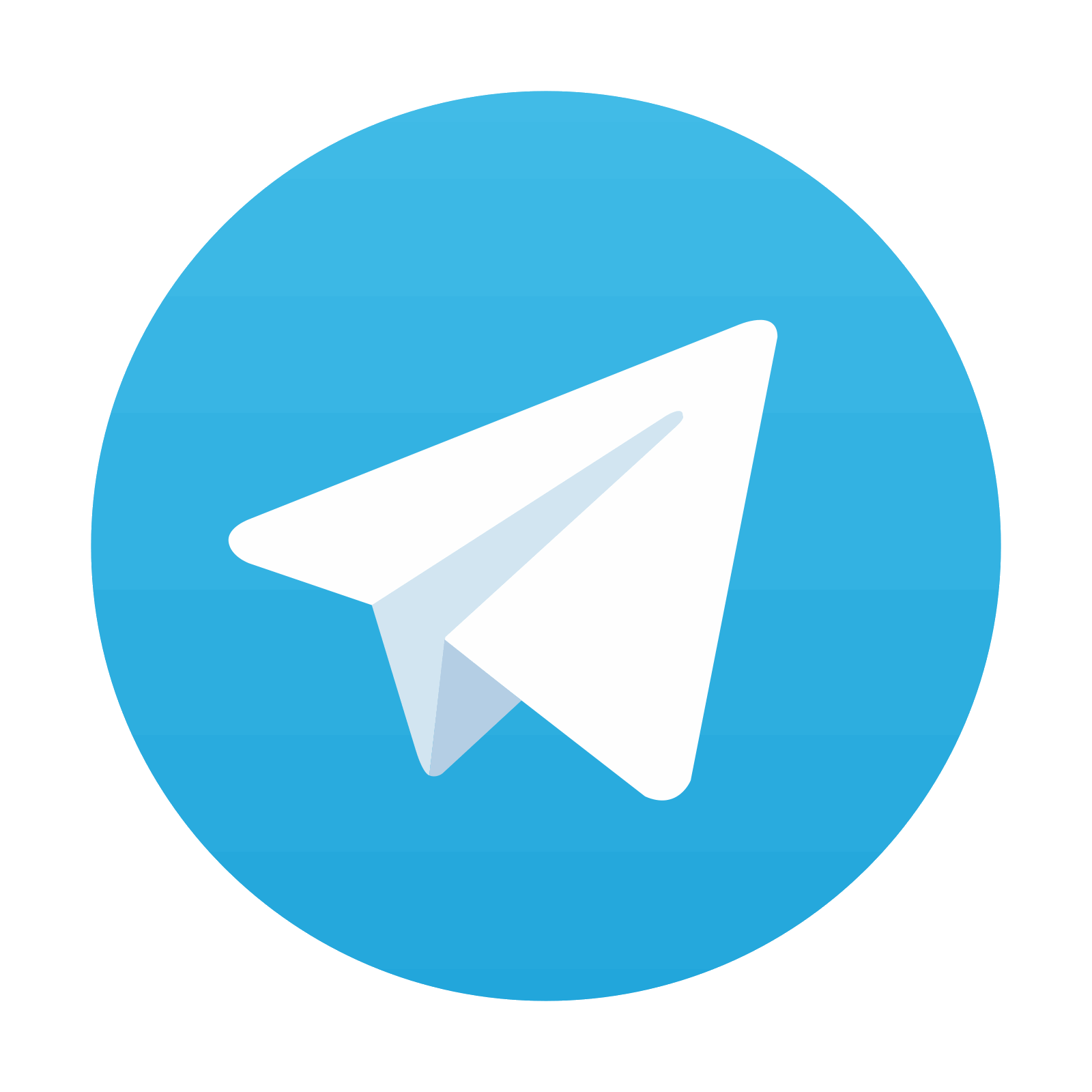
Stay updated, free articles. Join our Telegram channel
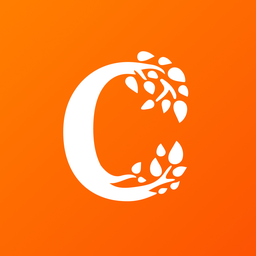
Full access? Get Clinical Tree
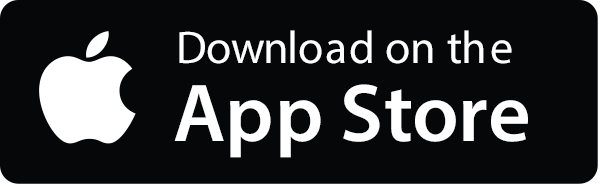
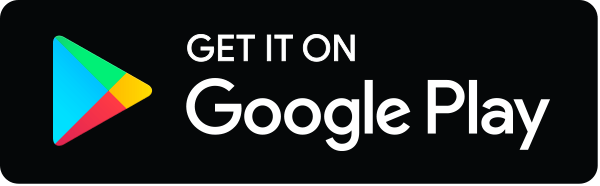