Abstract
In the United States, nearly 5% of the population has or has had cancer. Anesthetists frequently encounter patients in all stages of cancer and cancer treatment. This chapter highlights the short term and long term end-organ effects of common chemotherapeutics relevant to the anesthetist and reviews current concepts about how anesthetics may impact cancer and cancer survival.
Keywords
cardiotoxicity, pulmonary fibrosis, oxygen free radicals, malignancy, metastasis, monoclonal antibodies, immunotherapy, angiogenesis
Chapter Outline
Drugs That Cause DNA/RNA Damage
Drugs That Suppress Proliferation: Microtubule-Binding Agents
Molecular Therapies, Growth Inhibitors, and Targeted Therapies
How Anesthetics Might Affect Cancer
The concept of “immunosurveillance” describes a homeostatic balance whereby cells that undergo transformation into cancer cells are normally eliminated or kept in check by the immune system. In 1985 an autopsy study found that 36% of “normal” thyroid glands showed occult carcinomas, even though the incidence of clinically diagnosed thyroid cancer is one-thousandth of that rate in the same population. In 1987 another group found in situ breast carcinoma in 20% of autopsy examinations of women 20 to 54 years of age who died of unrelated causes. The expected incidence of clinically diagnosed breast cancer is on the order of about 0.1%. These studies found cancer cells 10 2 to 10 3 more frequently than cancer is manifest clinically. Malignant transformation of cells within the body is relatively common but clinical cases of cancer rarely result. The transformed cells are typically kept in check by immunosurveillance.
Once cancer is identified in a patient, treatment may include chemotherapy and radiation therapy aimed at killing the cancer cells, surgery to excise solid tumors, and immune therapy aimed at helping the immune system eliminate or at least modulate cancer cells. Surgical excision remains the best therapy for control of solid tumors (either with or without adjuvant therapies) and is what most often brings patients under the care of the anesthesiologist. Patients with nonsolid tumors (e.g., leukemias) may come to the operating room for biopsy or diagnostic staging. Patients also present for unrelated surgery regardless of the type of cancer and regardless of the stage of cancer therapy. Given the prevalence of newly diagnosed cancers each year (approximately 1,700,000 among a total population in the United States of 320,000,000 [0.5%]), along with the ever-increasing rate of survival of patients with a history of cancer (14,500,000 [4.5% of the population]), it is clear that anesthetists will frequently encounter patients in all stages of cancer and cancer treatment ( Table 38.1 ). They therefore have a responsibility to understand how cancer therapies might influence anesthetic management. In addition, researchers are exploring how anesthetic management might influence cancer. This chapter highlights end-organ effects of common chemotherapies and reviews current concepts about anesthetic effects on cancer. Both may prove important in determining the best possible anesthetic plan for the individual patient with cancer.
Primary Cancer | New Diagnoses a | Survivors b |
---|---|---|
Breast | 249,000 | 3,131,440 |
Lung | 224,000 | 430,000 |
Prostate | 181,000 | 2,975,970 |
Colorectal | 134,000 | 1,245,800 |
Bladder | 77,000 | 455,520 |
Melanoma | 76,000 | 528,860 |
Non-Hodgkin lymphoma | 73,000 | 569,800 |
Thyroid | 64,000 | 470,020 |
Kidney | 63,000 | 389,000 |
Leukemia | 60,000 | 177,940 |
Endometrial | 60,000 | 624,890 |
Pancreatic | 53,000 | |
All sites | 1,685,210 | 14,483,830 (4.5% of people in the United States) |
a Estimated number of patients with a new diagnosis of each cancer in 2016.
b Estimated number of patients living with a diagnosis of the specific cancer as of January 2014 (American Cancer Society, 2016). Available at: https://www.cancer.org/content/dam/cancer-org/research/cancer-facts-and-statistics/annual-cancer-facts-and-figures/2016/cancer-facts-and-figures-2016.pdf .
Chemotherapy
Using systemic medications to poison a cancer cell often results in collateral damage. The majority of chemotherapies target the cancer cell’s most distinctive feature, its rapid division and proliferation. However, cell division is not unique to the cancer cell. Disrupting cell division also affects normal functioning cells in involved and uninvolved internal organs, causing both acute and chronic injury after prior and/or cumulative exposures.
Understanding the mechanisms by which the different chemotherapeutics inflict injury on the target cancer cells helps predict and elucidate what injuries might occur in the rest of the body. For example, agents that interfere with DNA replication are effective against rapidly proliferating tumors but also harm areas where rapid cellular turnover is the norm (e.g., the intestinal mucosa and bone marrow). Agents that affect the activity of microtubules within the cell will affect microtubule function not only during cellular division, but also in the performance of other microtubule activities (e.g., the preparation and delivery of synaptic vesicles at the neuromuscular junction). Disrupting synaptic vesicles causes peripheral neuropathies. Finally, agents that kill rapidly dividing cells by generating oxygen free radicals also injure cells with high metabolic activity such as cardiac myocytes, leading to cardiomyopathies.
Conventional cancer drugs have a very narrow therapeutic index. They are highly toxic to both the targeted cancer cells and nontargeted cells. The “maximum tolerated dose” has the highest probability of decreasing tumor burden and is usually the prescribed dose. Anesthetists should therefore anticipate that their patients who have received chemotherapy have received near-toxic doses at which organ injury may occur.
In relation to surgery, chemotherapy can be administered (1) before surgical excision, as neoadjuvant therapy, to facilitate surgery by reducing tumor burden, killing micrometastases, and potentially decreasing the survivability of tumor cells liberated during surgical manipulation; (2) after surgical excision, as adjuvant therapy, in an attempt to eradicate clinically undetectable residual tumor burden; or (3) unrelated to surgical timing, as palliative therapy, to ameliorate patient symptoms.
Medications used to kill cancer cells can be divided into three broad categories. The first category directly damages DNA and/or ribonucleic acid (RNA), and includes alkylating agents, antimetabolites, antineoplastic antibiotics, and topoisomerase inhibitors. The second category suppresses proliferation of dividing cells by interfering with microtubule function. The third category includes targeted therapies, also known as precision medicine therapies. Targeted therapies are molecules that hinder specific cellular enzymes unique to or overexpressed in malignant cells. Targeted therapies can also be molecules made to attach to specific cell membrane antigens to mark the cell and facilitate recognition of the cancer by the immune system.
Drugs That Cause DNA/RNA Damage
Alkylating Agents
The prototypical alkylating agent, mechlorethamine (an analog of the chemical warfare agent mustard gas) is a simple molecule composed of an electrophilic backbone carrying an alkyl side chain ( Fig. 38.1 ). As an agent of war, mustard gas was a potent vesicant or blistering agent causing initial injury to exposed surfaces, including skin, eyes, and when inhaled, the lungs. Absorption would then lead to a drastic reduction in cell production in bone marrow and lymphatic tissue. During DNA replication, the alkyl group becomes covalently bonded to a DNA nucleotide (usually guanine) and physically interferes with subsequent transcription and cell division. On a molecular level this may be considered analogous to placing a set of handcuffs on rapidly dividing DNA ( Fig. 38.2 ). The alkylating agents are toxic in all phases of the cell cycle but are most lethal to rapidly proliferating tissues. Subcategories of alkylating agents ( Table 38.2 ) include nitrogen mustards (e.g., cyclophosphamide), nitrosureas (e.g., carmustine), alkyl sulfonates (e.g., busulfan), and platinum drugs (e.g., cisplatin).


Nitrogen Mustards | Nitrosureas | Triazines and Hydrazines | Ethyleneimines | Alkyl Sulfonates | Platinum Drugs a |
---|---|---|---|---|---|
Mechlorethamine | Arabinopyranosyl-N-methyl-N-nitrosourea (Aranose) | Dacarbazine | Thiotepa (Thioplex) | Busulfan | Cisplatin |
Chlorambucil | Carmustine (BCNU, BiCNU) | Temozolomide | Hexamethyl-melamine (HMM, altretamine, Hexalen) | Treosulfan | Carboplatin |
Cyclophosphamide (Cytoxan) | Chlorozotocin | Procarbazine | Mannosulfan | Oxaliplatin | |
Melphalan (Alkeran) | Ethylnitrosourea (ENU) | Nedaplatin | |||
Ifosfamide (Ifex) | Fotemustine | Satraplatin | |||
Trofosfamide | Lomustine (CCNU) | Triplatin tetranitrate | |||
Estramustine | Nimustine | ||||
N-nitroso-N-methylurea (NMU) | |||||
Ranimustine (MCNU) | |||||
Semustine | |||||
Streptozocin |
a Platinum-based agents do not literally alkylate but act like the other agents by binding to the guanine residue in DNA.
The alkylating agents are used to treat Hodgkin lymphoma, lymphosarcoma, leukemias, and bronchogenic sarcomas. Normal rapidly dividing cells—for example, bone marrow, intestinal mucosa, and hair follicles—are also killed along with the targeted tumor. The platinum-based chemotherapeutic agents are listed with the alkylating agents although they do not “alkylate” DNA (they do not have an alkyl group). They do act in the same manner by permanently binding to (hand-cuffing) guanine residues in DNA. Their main use is with testicular and ovarian cancers, bladder cancer, head and neck cancer, and small cell lung cancer. Oxaliplatin is used in metastatic colorectal cancer. The platinum-based agents additionally cause peripheral neuropathies and acute and chronic nephrotoxicity.
The alkylating agents have side effects mostly limited to bone marrow suppression and gastrointestinal tract effects, although late-onset pulmonary fibrosis is associated with long-term therapy (especially cyclophosphamide, busulfan, and the nitrosureas). Platinum-containing agents uniquely cause neuropathies and nephrotoxicity. Cyclophosphamide uniquely inhibits plasma pseudocholinesterase activity and can prolong the effect of succinylcholine. (Remifentanil, unlike succinylcholine, has an ester group that is metabolized by nonspecific blood and tissue esterases. Cyclophosphamide would not be expected to alter its metabolism.)
Antimetabolites
The antimetabolites are analogs of normal metabolites and inhibit cell growth and division ( Table 38.3 ). Methotrexate is a folic acid analog, 5-fluorouracil is an analog of uracil ( Fig. 38.3 ), and 6-mercaptopurine is a guanine analog. The analogs inhibit enzymes or cause them to synthesize aberrant molecules. They are frequently used in the treatment of colorectal, bladder, and pancreatic cancers as well as for leukemia. The antimetabolites affect all proliferating cells, causing side effects of immunosuppression, severe nausea and vomiting, ulcerative stomatitis and diarrhea, hemorrhagic enteritis, and potentially intestinal perforation, but there are few recognized long-term end-organ effects that alter anesthetic management.
Folate Analog | Pyrimidine Analogs | Purine Analogs |
---|---|---|
Methotrexate | 5-Fluorouracil | 6-Mercaptopurine |
Floxuridine | Azathioprine | |
Capecitabine | 6-Thioguanine | |
Gemcitabine | ||
Cytarabine (Ara-C) |

Antineoplastic Antibiotics
The antineoplastic antibiotics are almost wholly produced by Streptomyces bacteria. The Streptomyces are a genus of soil bacteria from which scientists harvest antibiotics (e.g., streptomycin, neomycin, tetracycline, chloramphenicol), antifungals (e.g., nystatin, amphotericin B), antiparasitics (ivermectin), and antitumor compounds (e.g., actinomycin D, bleomycin, and the anthracyclines). The antitumor compounds can be classified based on the primary mechanism by which they damage DNA. One group causes DNA alkylation, and another damages DNA by inhibiting topoisomerase II and by generating oxygen free radicals ( Fig. 38.4 ). The boundaries are not sharp and some crossover exists—for example the aminoquinones alkylate but also produce oxygen free radicals. The importance in the distinction lies in the cardiotoxicity of the agents that produce free radicals. The antitumor antibiotics that alkylate DNA show few long-term end-organ effects, whereas those that generate oxygen free radicals (e.g., the anthracyclines) are probably the most frequently cited and well-studied class of cardio-toxic anticancer agents. ( Table 38.4 )

Alkylate DNA | Alkylate and Produce Oxygen Radicals | Inhibit Topoisomerase Type II and Produce Oxygen Radicals a | Other Antineoplastic Antibiotics |
---|---|---|---|
Adozelesin | Mitomycin C | Anthracyclines b | Actinomycin D (Dactinomycin) |
Bizelesin | Streptonigrin | Anthracenediones c | Fostriecin |
Brostallicin | Anthrapyrazoles | Bleomycin | |
Carzelsin | Enediynes d | ||
CC-1065 | Miscellaneous | ||
Duocarmycins | |||
Mithramycin A | |||
Tallimustine |
a The underscored text represents subcategories of the antineoplastic antibiotics as listed in Table 38.5 .
b Acute cardiotoxicity includes arrhythmias, hypotension, and depression of contractility. Chronic toxicity results in cardiomyocyte apoptosis and can manifest a year or longer after exposure as congestive heart failure refractory to inotrope therapy.
c Developed to have less cardiotoxicity
d Produced by species other than Streptomyces . They are toxic but poorly selective for cancer cells, so current efforts are underway to conjugate these compounds with tumor-targeting molecules such as monoclonal antibodies.
Antineoplastic antibiotics are often used to treat breast and bladder cancers as well as leukemias and lymphomas. Many cause acute cardiotoxicity (arrhythmias, hypotension, decreased contractility) within a week of initiation of therapy as well as chronic cardiotoxicity that can develop late. The mechanism of the late cardiotoxicity is cardiomyocyte apoptosis largely owing to oxidative stress induced by reactive oxygen species (free radicals) generated intracellularly. Oxygen free radicals are generated within mitochondria and by a second pathway involving intracellular iron complexes. The heart is rich in mitochondria and has relatively poor ability to rid itself of oxygen free radicals. Cardiotoxicity is further amplified by topoisomerase inhibition in the cardiomyocyte that alters calcium channel activity. Acute cardiomyocyte injury from anthracyclines (e.g., doxorubicin (Adriamycin and daunorubicin) is associated with elevated serum troponin levels.
Acute doxorubicin toxicity is reversible, whereas subacute or chronic injury is irreversible. Acute, subacute, and chronic toxicity are related to the cumulative dose administered. The incidence of cardiomyopathy is about 4% when the cumulative dose is 500 to 550 mg/m 2 , 18% at 550 to 600 mg/m 2 , and 36% at greater than 600 mg/m 2 . Toxicity peaks at about 1 to 3 months after treatment and presents as biventricular congestive heart failure. Chest or mediastinal radiation, a previous history of cardiac injury, hypertension, and coadministration of other cardiotoxic chemotherapeutics (e.g., trastuzumab) increase the incidence of chronic cardiomyopathy. Late-onset dilated or restrictive cardiomyopathy can present years or decades after anthracycline treatment and can be first revealed during exposure to the myocardial-depressant effects of anesthetics. In addition, the biventricular failure has been shown to be poorly responsive to inotropes in animal models.
Analogs of the polycyclic aromatic antibiotics have been developed with the goal of decreasing the cardiotoxicity associated with anthracyclines ( Table 38.5 ). Other important antineoplastic antibiotics include the enediynes, actinomycin D (dactinomycin), and bleomycin. The enediynes include antibiotics that are produced by species other than Streptomyces . They damage DNA by oxidation (binding the DNA backbone and removing hydrogens), cleaving the DNA strands. They are highly toxic and their poor selectivity for cancer cells limits their current use. This might be overcome in the future by conjugating these compounds to tumor-targeting entities such as monoclonal antibodies (MAbs). Actinomycin D disrupts DNA transcription by binding to DNA at transcription initiation sites and preventing elongation by RNA polymerase. It can cause liver toxicity, specifically hepatic veno-occlusive disease and clotting disorders, that might not manifest for days after treatment ends.
Anthracyclines | Anthracenediones | Anthrapyrazoles | Enediynes |
---|---|---|---|
Doxorubicin (Adriamycin, Doxil, Myocet) | Mitoxantrone | Losoxantrone | Calicheamicin |
Daunorubicin | Ametantrone | Teloxantrone | Esperamicin |
Idarubicin | Pixantrone | Piroxantrone | Dynemicin |
Amrubicin | Kedarcidin | ||
Epirubicin | Maduropeptin | ||
Valrubicin | Neocarzinostatin | ||
Pirarubicin | Lidamycin | ||
Berubicin | |||
Carubicin | |||
Esorubicin | |||
Detorubicin | |||
Duborimycin | |||
Zorubicin | |||
Aclarubicin |
Bleomycin interacts with iron to produce oxygen free radicals. It is inactivated by the enzyme aminohydrolase (bleomycin hydrolase) that is found throughout the body except in the skin and lungs. Because of the scarcity of aminohydrolase in the skin, approximately 20% of patients receiving bleomycin therapy develop painless flagellate hyperpigmentation in their skin. In the lungs, where aminohydrolase activity is particularly low, alveolar epithelial cells accumulate bleomycin. Bleomycin injures the pulmonary endothelium, causing increased capillary permeability and edema, and the edema leads to even greater accumulation of bleomycin. Necrosis of the type I alveolar epithelium stimulates increased migration of macrophages, which recruit additional inflammatory cells that release oxygen radicals and stimulate fibroblast activity.
Exposure to high oxygen concentrations as part of operative anesthesia has been associated with potentiation of bleomycin pneumotoxicity and acute respiratory distress syndrome. A report of the deaths of five patients with acute respiratory distress syndrome–like symptoms after surgical procedures involving exposure to approximately 40% inspired oxygen concentrations 6 to 12 months after bleomycin treatment led to this association. This led to a recommendation to limit supplemental oxygen to <30%, or as necessary to maintain oxygen saturation as measured by pulse oximetry (Sp o 2 ) at 90% or above. Subsequent studies have identified other risk factors, including excessive intraoperative intravenous (IV) fluids and transfusions, preexisting renal failure, preexisting pulmonary disease, and tobacco use. Proximity to bleomycin infusion might be more important than high inspired oxygen concentrations, but minimizing inspired oxygen (<30% if possible) remains an important anesthetic guideline for reducing postoperative pulmonary complications.
When anesthetizing patients who have been exposed to antineoplastic antibiotics, four points should be considered:
- 1.
Subclinical asymptomatic cardiomyopathies can exist despite normal resting cardiac function. These can be unmasked by anesthesia.
- 2.
If congestive heart failure manifests, it is often refractory to inotrope therapy.
- 3.
Actinomycin D can cause hepatotoxicity and coagulation abnormalities.
- 4.
Bleomycin pulmonary fibrosis can be potentiated by exposure to elevated inspired oxygen, by excessive IV fluids, and by blood transfusions.
Topoisomerase Inhibitors
Topoisomerase enzymes are important for the proper activity of RNA and DNA polymerase. Polymerases separate DNA strands to transcribe base codes (whether to produce messenger RNA [mRNA] and proteins or to duplicate DNA in preparation for cellular division). As the polymerase separates segments of DNA, the remaining portion of the strands becomes more densely coiled ( Fig. 38.5 ). Topoisomerase enzymes cleave the hypercoiled segments of DNA, relax the DNA strands, and then reattach the cleaved ends, thereby allowing the transcription to progress. There are two classes of topoisomerase enzymes separated by whether they cleave one strand of the DNA (topoisomerase type I) or both strands (topoisomerase type II) as they relieve the hypercoiled state of the native DNA. Inhibitors of topoisomerase are specific to type I or type II.
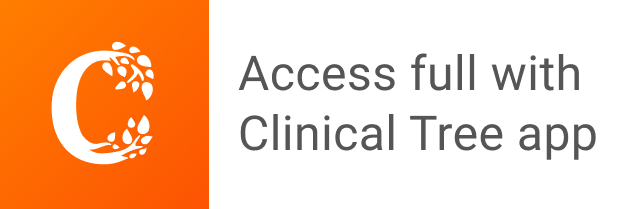