Abstract
Our understanding of the mechanisms involved in the action of general anaesthetics has increased considerably in recent times and is discussed below. This is followed by sections discussing intravenous and inhaled anaesthetic agents.
Our understanding of the mechanisms involved in the action of general anaesthetics has increased considerably in recent times and is discussed below. This is followed by sections discussing intravenous and inhaled anaesthetic agents.
Mechanisms of General Anaesthetic Action
Any mechanism of general anaesthetic action must be able to explain: loss of conscious awareness; loss of response to noxious stimuli (anti-nociceptive effect); and, perhaps most important of all, reversibility.
Anatomical Sites of Action
General anaesthetic agents affect both brain and spinal cord to account for physiological responses to nociception, loss of consciousness and inhibition of explicit memory. Auditory and sensory evoked potential data implicate the thalamus as the most likely primary target, but secondary sites such as the limbic system (associated with memory) and certain cortical areas are also important. Halogenated volatile anaesthetics appear to have a greater influence on the spinal cord than do the intravenous agents.
Molecular Theories
At the beginning of the twentieth century, Overton and Meyer independently described the linear correlation between the lipid solubility of anaesthetic agents and their potency (see Figure 9.1). This correlation was so impressive, given the great variation in structure of these agents, that it suggested a non-specific mechanism of action based on this physicochemical property. Later interpretation pointed out that any highly lipophilic area was a potential site of action, with cell membranes being the most likely contender, given the high concentration of lipids. There are problems with a unified theory based on lipid interactions: some general anaesthetics, such as ketamine, are extreme outliers, and the stereoisomers R-etomidate and S-etomidate have identical lipid solubility but only R-etomidate has anaesthetic properties.
Membrane Lipids
There are several potential lipophilic sites in cell membranes, including the lipid bilayer itself and the annular lipids surrounding ionic channels.
Initially it was suggested that anaesthetic agents could penetrate the bilayer and alter the molecular arrangement of the phospholipids, which led to expansion of the membrane and disruption of the function of membrane-spanning ionic channels. Calculations identifying the volume of anaesthetic agent required to expand membranes led to a ‘critical volume hypothesis’. Against such a theory, a 1°C rise in temperature increases membrane thickness to a similar extent as that seen with volatile agents, yet increased temperature does not enhance anaesthesia – the opposite is true.
A further theory suggested anaesthetic agents act at specific lipid site(s). The composition of phospholipids in the immediate vicinity of ion channels is different from that of the general lipid bilayer. This proposed disruption of annular lipids associated with specific ion channels led to the perturbation theory.
A rapid advance in receptor protein identification within the central nervous system (CNS) has led to newer theories based on interactions with specific proteins. It now seems likely that the correlation between potency and lipid solubility reflects the lipophilic nature of specific protein-based binding site(s).
Protein Site(s) of Action
Ligand-gated ionic channels are more sensitive to the action of general anaesthetics than are voltage-gated channels. The interaction at inhibitory (GABAA and glycine) and excitatory (neuronal nicotinic and NMDA) channels have all been studied. Table 9.1 summarises the relative activity of a number of agents at these receptors.
Inhibitory neurotransmitters | Excitatory neurotransmitters | |||
---|---|---|---|---|
GABAA | Glycine | NMDA | Neuronal nACh | |
Propofol |
|
| 0 | − |
Thiopental |
|
| 0 | − |
R-etomidate |
| 0 | 0 | 0 |
S-etomidate | 0 | 0 | 0 | 0 |
Ketamine | 0 | 0 | − | 0 |
Isoflurane |
|
| 0 | − |
Nitrous oxide | 0 | 0 | − | 0 |
Xenon | 0 | 0 | − | 0 |
+: enhances effect of neurotransmitter; −: reduces effect of neurotransmitter; 0: no effect on neurotransmitter. nACh: central nicotinic acetylcholine receptor.
GABAA Receptor
The GABAA receptor, like the nicotinic acetylcholine receptor, belongs to the pentameric family of ligand-gated ion-channel receptors. It has binding sites for GABA associated with α subunits and modulatory sites at the α/γ interface for benzodiazepines and on the β subunit for etomidate, barbiturates, propofol and volatile agents (see Figure 9.2). The stereospecificity of the action of etomidate, presented as an enantiopure preparation of the R(+) isomer, supports the protein-based action of anaesthetics. The S(−) form of etomidate is clinically inactive and at the GABAA receptor there is a 30-fold difference in activity.
Figure 9.2 The GABAA receptor complex, from above. The grey triangles show the two agonist sites for gamma amino butyric acid (GABA). Diazepam, temazepam and midazolam are agonists and flumazenil is an antagonist at the benzodiazepine site (grey circle). Propofol, etomidate, barbiturates and halogenated volatile agents are agonists at the general anaesthetic site. Both sites produce positive allosteric modulation.
Anaesthetics increase channel opening time, so allowing for increased chloride entry resulting in hyperpolarisation. The effect is seen for etomidate, propofol and barbiturates, as well as halogenated volatiles. Etomidate is selective for the GABAA receptor but propofol will also increase glycine channel opening time and is inhibitory at neuronal nicotinic and 5HT3 receptors. The site(s) on the GABAA receptor associated with anaesthetic action are associated with the β subunit and are distinct from the benzodiazepine receptor site. There are at least 30 types of GABAA receptor, each with different subunit composition; β2 and β3 subunits are more sensitive to the effects of etomidate than is the β1 subunit.
Glycine Receptor
The major inhibitory transmitter in the spinal cord and brain stem is glycine, which is associated with a chloride channel similar to the GABAA receptor. The volatile anaesthetics all markedly potentiate the action of glycine, although there is no evidence of stereoselectivity. It has been suggested that the spinal cord is an important site of action for volatile rather than intravenous anaesthetic agents. Efficacy here correlates more with immobility than awareness.
NMDA Receptor
Neuronal signalling may also be reduced by inhibition of excitatory pathways. The NMDA receptor is involved in long-term signal potentiation associated with learning and memory; it is activated by glutamate, modulated by magnesium and inhibited in a non-competitive manner by ketamine, nitrous oxide and xenon. This glutamate-mediated mechanism represents an additional pathway for anaesthesia. Other anaesthetic agents, such as barbiturates, can reduce the effectiveness of glutamate but at a lower potency than for inhibition of GABAA receptor function.
Intravenous Anaesthetic Agents
Intravenous anaesthetics have been defined as agents that will induce loss of consciousness in one arm–brain circulation time.
The introduction of barbiturates in the 1930s was a significant advance in anaesthesia. Their rapid onset and relatively short duration of action made them different from previously used agents. Hexobarbitone was introduced first, followed by thiopental and subsequently methohexitone. Phencyclidine (angel dust) was withdrawn due to serious psychotomimetic reactions, but the chemically related compound ketamine is still used. The imidazole ester, etomidate, is useful due to its cardiovascular stability but side effects limit its use. The phenolic derivative propofol has become the most popular agent in recent years due to its ready-to-use formulation, favourable recovery profile and use in target-controlled infusions (TCIs). Steroidal compounds have also been used; however, poor solubility (pregnalolone) together with an association with anaphylactic reactions (due to cremophor EL – used to solubilise the steroid althesin) has led to their demise.
The Ideal Intravenous Anaesthetic Agent
Were an ideal intravenous anaesthetic agent to exist, it should have the following properties:
rapid onset (mainly unionised at physiological pH)
high lipid solubility
rapid recovery, no accumulation during prolonged infusion
analgesic at sub-anaesthetic concentrations
minimal cardiovascular and respiratory depression
no emetic effects
no pain on injection
no excitation or emergence phenomena
no interaction with other agents
safe following inadvertent intra-arterial injection
no toxic effects
no histamine release
no hypersensitivity reactions
water-soluble formulation
long shelf-life at room temperature
minimal environmental impact.
The currently used agents are discussed below under the following headings:
Barbiturates
All barbiturates are derived from barbituric acid, which is the condensation product of urea and malonic acid (see Figure 9.3). When oxygen is exchanged for sulfur at the C2 position, oxybarbiturates become thiobarbiturates.
Barbiturates are not readily soluble in water at neutral pH. Their solubility depends on transformation from the keto to the enol form (tautomerism), which occurs most readily in alkaline solutions (see Figure 9.4). In general, thiobarbiturates are very lipid-soluble, highly protein-bound and completely metabolised in the liver. In contrast, the oxybarbiturates are less lipid-soluble, less protein-bound, and some are excreted almost entirely unchanged in the urine.
Thiopental
Thiopental is the sulfur analogue of the oxybarbiturate pentobarbital.
Presentation
Thiopental is formulated as the sodium salt and presented as a pale yellow powder. The vial contains sodium carbonate (Na2CO3, 6% by weight) and nitrogen in place of air. These two measures are designed to improve solubility of the solution by the following mechanisms:
1. Sodium carbonate reacts with water in the following manner:

The result is a strongly alkaline solution (pH 10.5) favouring the water-soluble enol form which is more desirable as a preparation.
2. Air contains small amounts of carbon dioxide. Were this to be present and react with water it would tend to release bicarbonate and hydrogen ions which in turn would result in a less alkaline solution. As a result, more thiopental would be in the less water-soluble keto form. Nitrogen is used in place of air to prevent this.
The 2.5% solution is stable for many days and should be bacteriostatic due to its alkaline pH.
Uses
Apart from induction of anaesthesia (3–7 mg.kg−1 intravenously) thiopental is occasionally used in status epilepticus. At sufficient plasma concentrations (most easily maintained by continuous infusion) thiopental produces an isoelectric EEG, confirming maximal reduction of cerebral oxygen requirements. Inotropic support may be required to maintain adequate cerebral perfusion at these doses. It has previously been used rectally, although it has a slow onset via this route.
Effects
Cardiovascular – there is a dose-dependent reduction in cardiac output, stroke volume and systemic vascular resistance that may provoke a compensatory tachycardia. These effects are more common in patients that are hypovolaemic, acidotic and have reduced plasma protein binding.
Respiratory – respiratory depression is dose-dependent. It may produce a degree of laryngospasm and bronchospasm.
Central nervous system – a single dose will rapidly induce general anaesthesia with a duration of about 5–10 minutes. There is a reduction in cerebral oxygen consumption, blood flow, blood volume and cerebrospinal fluid pressure. When used in very low doses it is antanalgesic.
Renal – urine output may fall, not only as a result of increased antidiuretic hormone release secondary to CNS depression, but also as a result of a reduced cardiac output.
Severe anaphylactic reactions – these are seen in approximately 1 in 20,000 administrations of thiopental.
Porphyria – thiopental may precipitate an acute porphyric crisis and is therefore absolutely contraindicated in patients with porphyria. The following drugs may also precipitate an acute porphyric crisis:
other barbiturates
etomidate
halothane
cocaine
lidocaine and prilocaine (bupivacaine is safe)
clonidine
metoclopramide
hyoscine
diclofenac
ranitidine.
Figure 9.5 Thiopental in plasma. Only 12% is immediately available as non-protein-bound and unionised drug.
Kinetics
Thiopental is predominantly bound by plasma proteins with only 20% free and unbound within the plasma. Its pKa of 7.6 means that 60% of the free form is unionised (i.e. 12% of the total drug in the plasma). This relatively small fraction is immediately available to achieve its effects. Despite this it has a rapid onset due to its high lipid solubility and the large cardiac output that the brain receives. In addition, a dynamic equilibrium exists between protein-bound and free drug. Critically ill patients tend to be acidotic and have reduced plasma protein binding, resulting in a greater fraction of drug in the unionised form and fewer plasma protein binding sites, so that a smaller administered dose of thiopental is required to induce anaesthesia. Non-steroidal anti-inflammatory drugs may also reduce available protein binding sites and increase the fraction of free drug.
Rapid emergence from a single bolus dose is due to rapid initial distribution into tissues, not metabolism. A tri-exponential decline is seen representing distribution to well-perfused regions (brain, liver) followed by muscle and skin. The final decline is due to hepatic oxidation mainly to inactive metabolites (although pentobarbital is also a metabolite). When given as an infusion its metabolism may become linear (zero-order, see p. 62) due to saturation of hepatic enzymes. The hepatic mixed-function oxidase system (cytochrome P450) is induced after a single dose.
Intra-arterial Injection
If 2.5% thiopental at p. 10.5 is injected into arterial blood with a pH of 7.4 the tautomeric equilibrium swings away from the enol towards the keto form, resulting in a less water-soluble solution. This in turn leads to the precipitation of thiopental crystals which become wedged into small blood vessels leading to ischaemia and pain. Treatment should begin immediately and may include intra-arterial injection of papaverine or procaine, analgesia, sympathetic block of the limb and anticoagulation.
Thiopental does not precipitate when injected intravenously as it is continually diluted by more venous blood. Peri-vascular injection is painful and may cause serious tissue necrosis if large doses extravasate.
Non-barbiturates
Propofol
Presentation
This phenolic derivative (2,6 diisopropylphenol, see Figure 9.6) is highly lipid-soluble and is presented as a 1% or 2% lipid–water emulsion due to poor solubility in water. There are a number of generic formulations which differ in their excipients although all contain soya bean oil, egg lecithin and glycerol. The addition of medium chain triglycerides has been used to reduce the proportion of free propofol and the incidence of pain during administration. It is a weak organic acid with a pKa of 11 so that it is almost entirely unionised at p. 7.4.
Figure 9.6 Chemical structure of some intravenous anaesthetics.
Uses
Propofol is used for the induction and maintenance of general anaesthesia and for sedation of ventilated patients in intensive care. The induction dose is 1–2 mg.kg−1 while a plasma concentration of 4–8 µg.ml−1 will maintain anaesthesia.
Effects
Cardiovascular – the systemic vascular resistance falls, resulting in a drop in blood pressure. A reflex tachycardia is rare and propofol is usually associated with a bradycardia, especially if administered with an opioid. Sympathetic activity and myocardial contractility are also reduced.
Respiratory – respiratory depression leading to apnoea is common. It is rare to observe cough or laryngospasm following its use and so it is often used to facilitate placement of a supraglottic airway.
Central nervous system – excitatory effects have been associated with propofol in up to 10% of patients. They probably do not represent true cortical seizure activity; rather they are the manifestation of subcortical excitatory–inhibitory centre imbalance. The movements observed are dystonic with choreiform elements and opisthotonos. Propofol has been used to control status epilepticus.
Gut – limited evidence exists to suggest that propofol possesses antiemetic properties following its use for induction, maintenance or in subhypnotic doses post-operatively. Antagonism of the dopamine D2 receptor is a possible mechanism.
Pain – injection into small veins is painful but may be reduced if lidocaine is mixed with propofol or if the affected limb is raised to facilitate venous drainage.
Metabolic – a fat overload syndrome, with hyperlipidaemia, and fatty infiltration of heart, liver, kidneys and lungs can follow prolonged infusion, see below, ‘Toxicity’.
Kinetics
Propofol is extensively bound to both plasma proteins (mainly albumin) and erythrocytes, with only 1–2% remaining free in the plasma. It readily crosses the blood–brain barrier leaving the cardiac output and speed of administration as keys to the speed of induction. Following bolus administration, its duration of action is short due to the rapid decrease in plasma levels as it is distributed to well-perfused tissues. It has the largest volume of distribution of all the induction agents at 4 l.kg−1 (see Table 9.2) due to it’s high lipid solubility. Even after long durations of administration its offset is still relatively fast as redistribution from these fat stores is slow compared with the rates of metabolism and excretion (cf. context-sensitive half-time, p. 78).
Dose (mg.kg−1) | Volume of distribution (l.kg−1) | Clearance (ml.kg−1.min−1) | Elimination half-life (h) | Protein binding (%) | Metabolites | |
---|---|---|---|---|---|---|
Thiopental | 3–7 | 2.5 | 3.5 | 6–15 | 80 | active |
Propofol | 1–2 | 4.0 | 30–60 | 5–12 | 98 | inactive |
Ketamine | 1–2 | 3.0 | 17 | 2 | 25 | active |
Etomidate | 0.3 | 3.0 | 10–20 | 1–4 | 75 | inactive |
Metabolism is mainly hepatic, with approximately 70% being conjugated to propofol glucuronide while 30% undergoes a Phase 1 reaction to 4-hydroxypropofol within CYP2B6 (and CYP2C9). From here it is conjugated to form 4-hydroxypropofol-sulphate, 4-hydroxypropofol-glucuronide and 1-hydroxypropofol-glucuronide. All the conjugated forms are then excreted in the urine, alongside a tiny fraction (less than 1%) of unchanged propofol. None of the major metabolites (Figure 9.7) have hypnotic activity. The liver is very efficient at propofol metabolism with a blood extraction ratio of 90% so that reductions in hepatic blood flow lead to reductions in its metabolism. Its clearance (of about 2.2 l.min−1) exceeds hepatic blood flow, indicating some extra-hepatic metabolism, which may reach up to 40%. In addition, the kidneys are responsible for up to 30% of its metabolism. Owing to this high clearance, plasma levels fall more rapidly than those of thiopental following the initial distribution phase. Its terminal elimination half-life is 5–12 hours although it has been suggested that when sampling is performed for longer than 24 hours the figure approaches 60 hours and may reflect the slow release of propofol from fat. During prolonged infusion its context-sensitive half-time increases, although where the infusion has been titrated carefully, waking may still be relatively rapid.
Figure 9.7 The metabolic pathway of propofol
Toxicity
The term Propofol Infusion Syndrome (PRIS) has been used to describe an acute refractory bradycardia, leading to asystole, in the presence of at least one of the following features: metabolic acidosis (BE > –10 mmol.l−1), rhabdomyolysis or myoglobinuria, lipaemic plasma or an enlarged/fatty liver. It was originally described in children but has also been reported in adults during periods of sedation in Intensive Care Units (ICUs).
It typically occurs after 3 days of propofol-based sedation, and is associated with an increased mortality compared with patients being sedated with other drugs. It presents most commonly with new onset metabolic acidosis and cardiac dysfunction which is pre-empted by ECG changes which are similar (but not limited) to those seen in Brugada syndrome. Direct muscle necrosis with creatine kinase (CK) levels of > 10,000 units.l−1 may occur. Animal and human models have demonstrated that propofol interferes with oxidative phosphorylation, mitochondrial energy production and electron transfer in myocytes. This leads to a mismatch between energy demand and utilisation and ultimately to muscle necrosis.
Where propofol sedation is used it should not exceed 4 mg.kg−1.hr−1 for more than 48 hours and should be done with regular CK, lactate and triglyceride monitoring. Patients with sepsis, burns, trauma, pancreatitis and neurological injuries are at high risk. It is contraindicated in patients of 16 years and younger for sedation in ICU.
Following a single case of pruritis in a patient with egg allergy, and a subsequent report of two patients who developed bronchospasm after propofol administration, it was suggested that soybean oil and egg yolk lecithin might have been responsible, despite an absence of allergy testing. A further case of hypotension and bronchospasm in a child, known to be allergic to egg and peanut (but not soy), gave further credence to this narrative, also in the absence of allergy testing. A more thorough analysis of much larger patient cohorts allergic to egg, soy and peanut has concluded that propofol is safe in this group. However, the picture is more complicated in children where there is no persuasive evidence that propofol is unsafe in those allergic to soy or peanut, but for children allergic to egg it is advised that more evidence is required for propofol to be used with confidence.
Propofol does not appear to cause any adverse effects when given intra-arterially, although onset of anaesthesia is delayed. Caution is required when using a peripheral intravenous cannula whose tip does not lie in a superficial vein. If its lumen is not intravascular, deep subcutaneous injection of propofol is not especially painful and may only be recognised when induction fails. Erythema, pain and swelling over the subsequent 24 hours appears to be dose-dependent.
Environmental Impact
Unlike the inhaled agents, propofol has no direct global warming potential. Its environmental footprint relates to production, transport and the electricity used when delivered via a syringe pump. Consequently, its global warming potential is four orders of magnitude less when compared to the inhaled agents. It is said to have ‘moderate persistence’ in the environment where it is toxic to aquatic organisms, but the overall risk is low. In order to prevent environmental contamination, unused propofol should not be discarded down the sink, but disposed of in containers destined for incineration.
Ketamine
Ketamine is a phencyclidine derivative.
Presentation and Uses
Ketamine is presented as a racemic mixture or as the single S(+) enantiomer, which is two to three times as potent as the R(–) enantiomer. It is soluble in water forming an acidic solution (pH 3.5–5.5). Three concentrations are available: 10, 50 and 100 mg.ml−1, and it may be given intravenously (1–2 mg.kg−1) or intramuscularly (5–10 mg.kg−1) for induction of anaesthesia. Intravenous doses of 0.2–0.5 mg.kg−1 may be used to facilitate the positioning of patients with fractures before regional anaesthetic techniques are performed. It has been used via the oral and rectal route for sedation and also by intrathecal and epidural routes for analgesia. Historically, its use has been limited by unpleasant side effects. It has gained popularity at low doses in combination with other analgesics and may be particularly useful in those with an element of chronic pain.
Effects
Cardiovascular – ketamine is unlike other induction agents in that it produces sympathetic nervous system stimulation, increasing circulating levels of adrenaline and noradrenaline. Consequently heart rate, cardiac output, blood pressure and myocardial oxygen requirements are all increased (see Table 9.3). However, it does not appear to precipitate arrhythmias. This indirect stimulation masks the mild direct myocardial depressant effects that racemic ketamine would otherwise exert on the heart. S(+) ketamine produces less direct cardiac depression in vitro compared with R(−) ketamine. In addition, while racemic ketamine has been shown to block ATP-sensitive potassium channels (the key mechanism of ischaemic myocardial preconditioning), S(+) ketamine does not, which therefore must be considered advantageous for patients with ischaemic heart disease.
Respiratory – the respiratory rate may be increased and the laryngeal reflexes relatively preserved. A patent airway is often, but not always, maintained, and increased muscle tone associated with the jaw may precipitate airway obstruction. It causes bronchodilation and may be useful for patients with asthma.
Central nervous system – ketamine produces a state of dissociative anaesthesia that is demonstrated on EEG by dissociation between the thalamocortical and limbic systems. In addition, intense analgesia and amnesia are produced. The α rhythm is replaced by θ and δ wave activity. Ketamine is different from other intravenous anaesthetics as it does not induce anaesthesia in one arm–brain circulation time – central effects becoming evident 90 seconds after an intravenous dose. Vivid and unpleasant dreams, hallucinations and delirium may follow its use. These emergence phenomena may be reduced by the concurrent use of benzodiazepines or opioids. S(+) ketamine produces less intense although no less frequent emergence phenomena. They are less common in the young and elderly and also in those left to recover undisturbed. Cerebral blood flow, oxygen consumption and intracranial pressure are all increased. Muscle tone is increased and there may be jerking movements of the limbs. A nasal spray of S(+) ketamine has been approved by the FDA for the treatment of resistant depression.
Analgesia – when given by infusion at doses low enough to avoid side effects ketamine may still retain useful analgesic effects and reduce morphine consumption.
Gut – nausea and vomiting occur more frequently than after propofol or thiopental. Salivation is increased requiring anticholinergic premedication.
Bladder – non-prescription, high-dose use has been associated with severe interstitial cystitis that may require cystectomy.
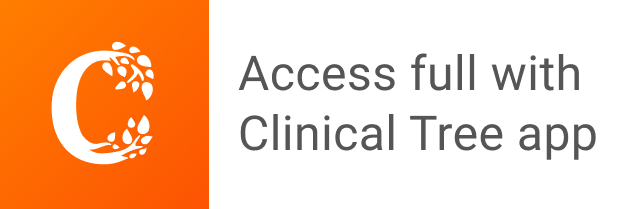