Abstract
Water is the most abundant component of the human body. On average, 60% of the body is composed of water (this value varies with sex, body habitus and age). Body water is distributed between the two major body compartments: intracellular and extracellular. For the average 70‑kg man.
How is water distributed in the body?
Water is the most abundant component of the human body. On average, 60% of the body is composed of water (this value varies with sex, body habitus and age). Body water is distributed between the two major body compartments: intracellular and extracellular. For the average 70‑kg man:
Total body water is 42 L (60% of 70 kg, where 1 kg of water has a volume of 1 L).
Approximately two-thirds of body water is intracellular fluid (ICF); that is, 28 L.
Approximately a third of body water is extracellular fluid (ECF); that is, 14 L. Of the ECF:
– Approximately a fifth is intravascular fluid; that is, plasma volume is around 3 L.
– A smaller proportion (around 1 L) is transcellular fluid, such as cerebrospinal fluid, ocular fluid, synovial fluid.
– The remainder is interstitial fluid (around 10 L), the fluid that occupies the spaces between cells. It is within this fluid that capillaries and cells exchange nutrients and waste products.
How is the volume of water within different body compartments measured?
Body fluid compartments are typically measured using an indicator-dilution method. A known quantity of an indicator substance is administered, allowed to equilibrate across the body compartment of interest and its concentration measured. The key to this method is an understanding of the barriers between body compartments and selection of the correct indicator and its permeability properties, ensuring that it selectively accesses the relevant compartment:
Calculation of plasma volume requires an indicator that, once infused into the circulation, cannot cross the capillary endothelium, such as radio-labelled albumin.
Calculation of ECF volume requires an indicator that is able to cross the capillary endothelium but cannot gain access to the ICF by crossing the cells’ phospholipid bilayer, such as thiosulphate.
Calculation of total body water requires an indicator that can distribute across all body fluid compartments. The indicator must be able to cross both the capillary endothelium and the cells’ phospholipid bilayer. Deuterated water (2H2O) is often used.
The volumes of the ICF and interstitial fluid compartments cannot be directly measured. Instead, interstitial fluid volume can be calculated from the difference between ECF and plasma volumes. Likewise, ICF volume can be calculated from the difference of total body water and ECF volume.
How is plasma volume regulated?
The maintenance of intravascular volume is a problem familiar to anaesthetists:
Too low a blood volume results in reduced venous return, reduced cardiac preload and therefore reduced cardiac output, systemic hypotension and organ ischaemia.
Too high a blood volume is also potentially harmful: excessive preload stretches cardiac myocytes, which may precipitate left ventricular failure, and induces hypertension, which damages organs such as the kidneys, heart and retina.
The body cannot control the volume of ECF by moving water directly between compartments: there are no water pumps in the body. Na+ is the major cation of the ECF; together with its conjugate anion, Na+ accounts for over 90% of the body’s osmotic activity. ECF volume is therefore regulated by controlling the movement of Na+ and thus water. Extracellular Na+ concentration is a balance of:
Na+ intake – dietary or intravenous;
Extra-renal Na+ loss – for example, sweating, faeces;
Renal Na+ excretion.
The kidney is essentially responsible for plasma Na+ regulation, as it can significantly vary its Na+ excretion. The kidney therefore regulates plasma volume and thus ECF volume:
What is osmolarity? How does it differ from molarity?
Osmolarity is a measure of the number of dissolved osmotically active particles per unit volume of a solution.
Osmolarity is defined as the number of osmoles per litre of solution, where osmoles denotes the number of moles of particles that are able to exert an osmotic pressure.
In contrast, molarity is the number of moles of solute dissolved per litre of solution; that is, the concentration.
For example, 1 mol of sodium chloride dissolved in water completely dissociates into Na+ and Cl‾ ions, thereby yielding 2 Osmol of osmotically active particles. In comparison, 1 mol of glucose dissolves in water but cannot dissociate into ions, thus yielding only 1 Osmol of osmotically active particles.
Plasma osmolarity can be estimated at the bedside by summing the concentrations of the most common osmolytes:
Key equation: estimated plasma osmolarity
Plasma osmolarity = 2[Na+] + 2[K+] + [glucose] + [urea], where [X] (mmol/L) is the concentration of substance X.
In this estimate, the concentrations of Na+ and K+ are doubled to account for their conjugate anions (some of which may not be routinely measured in the laboratory).
What is osmolality? How does it differ from osmolarity?
Osmolality is a measure of the number of dissolved osmotically active particles per unit mass of a solution. The problem with using osmolarity results from changes in volume of solvent (water) with changes in temperature and with the introduction of solute. As solvent mass does not vary with temperature, osmolality is independent of temperature and the weight of the solute. Osmolality is measured in the laboratory by a method based on the depression of the freezing point of the solution.
Osmolarity and osmolality are often used interchangeably, as numerically they are similar in normal patients. However, in certain situations, an osmolar gap exists – a difference between the measured osmolality and the calculated osmolarity:
Key equation: osmolar gap
Osmolar gap = osmolality – osmolarity
An osmolar gap indicates the presence of additional unmeasured osmotically active particles in the plasma that are not included in the estimation of osmolarity. Clinically important causes of a high osmolar gap include alcohol intoxication, hypertriglyceridaemia and methanol and ethylene glycol poisoning.
Why is it so important that plasma osmolarity is regulated?
Osmolarity must be tightly regulated, as it alters the fluid tonicity; tonicity refers to the response of intracellular water to the osmolarity of the surrounding ECF:
If the ECF is isotonic, the cells stay the same size.
If the ECF is hypertonic, the cells shrink due to the extracellular movement of water by osmosis.
If the ECF is hypotonic, the cells swell due to the intracellular movement of water by osmosis.
Infusion of sterile water into a peripheral vein acutely lowers the osmolarity of venous blood. The circulating red blood cells (RBCs) find themselves surrounded by hypotonic solution. Water moves into the RBCs, causing them to swell and potentially haemolyse. An RBC can only accommodate a limited amount of extra water before haemolysis occurs. For this reason, infusions of 5% dextrose are used in place of sterile water:
5% dextrose is approximately isotonic (osmolarity of 278 mOsmol/L compared with plasma osmolarity of 285–295 mOsmol/L).
Once the glucose is metabolised, it is as if free water has been infused, but without the acute drop in osmolarity.
Severe hyponatraemia is usually accompanied by a fall in plasma osmolarity, which results in generalised cell swelling. This is particularly dangerous in the brain, which is confined within the rigid structure of the skull. Cerebral oedema may result in raised intracranial pressure, seizures, altered consciousness and brainstem herniation.
How is plasma osmolarity controlled in the body?
Plasma osmolarity is controlled by means of a feedback loop. Like all feedback loops in the body, there are:
Sensors. Osmoreceptors are located in the organum vasculosum of the lamina terminalis and subfornical organ within the hypothalamus.
Control centre. The hypothalamus interprets the response from the osmoreceptors. Osmoreceptors are extremely sensitive: they can detect as little as a 1% change in plasma osmolarity. The normal set point of plasma osmolarity is 285–295 mOsmol/L.
Effectors. The hypothalamus responds to a rise in plasma osmolarity in two ways:
– Stimulating thirst: oral water intake is increased.
– Reducing water excretion by the kidney: antidiuretic hormone (ADH; also known as arginine-vasopressin) is synthesised by the hypothalamus and transported to the posterior lobe of the pituitary gland through nerve axons, where it is stored in granules. In response to an increase in plasma osmolarity, the hypothalamus signals the posterior lobe of the pituitary to secrete ADH into the systemic circulation. ADH acts at the collecting ducts of the kidney, increasing the reabsorption of free water and thus reducing plasma osmolarity.
Similarly, if plasma osmolarity were to fall, the hypothalamus reduces the sensation of thirst and inhibits the secretion of ADH, thereby increasing the amount of water excreted by the kidney.
What is the mechanism by which ADH acts at the kidney?
ADH regulates the water permeability of the collecting ducts in the kidney (Figure 69.1):
Normally, the luminal wall of the collecting ducts is impermeable to water. Therefore, any renal filtrate that passes through the distal convoluted tubule (DCT) and into the collecting duct is destined for excretion as urine.
ADH binds to V2 receptors in the collecting ducts, which, through a cyclic AMP second messenger system, results in a water channel (aquaporin 2) being inserted in the luminal walls of the collecting ducts.
This makes the collecting ducts permeable to water, which flows along an osmotic gradient, from an area of low osmolarity (the filtrate) to an area of high osmolarity (the renal medulla). A reduced volume of water is therefore excreted in the urine.
The key to this process is the extremely high osmolarity of the renal medulla, generated by the loops of Henle (LOH) and its countercurrent mechanism, and through urea cycling.
How is the high osmolarity of the renal medulla generated?
As discussed in Chapter 67, the LOH is located in the renal medulla, where it connects the proximal convoluted tubule (PCT) to the DCT. It is composed of three functional parts:
The thin descending limb, which is permeable to water but relatively impermeable to ions and urea;
The thin ascending limb, which is permeable to ions and urea, but impermeable to water, potentially leading to a separation of ion and water movement;
The thick ascending limb, which is also impermeable to water, but additionally moves ions via secondary active transport involving luminal Na+/K+/2Cl‾ co-transporters powered by secondary active transport from the basolateral Na+/K+-ATPase.
Figure 69.2 summarises alterations in the renal filtrate as it passes along the LOH:
In the PCT, water is reabsorbed in conjunction with ions, amino acids and glucose. The osmolarity of the fluid entering the descending limb of the LOH is therefore roughly the same as plasma osmolarity; that is, 300 mOsmol/L (1).
When the filtrate reaches the thick ascending limb of the LOH, the Na+/K+/2Cl‾ co-transporter moves Na+, K+ and Cl‾ from the filtrate to the medullary interstitium (2). As the ascending limbs are impermeable to water:
– The filtrate entering the DCT becomes hypo-osmolar.
– The renal medullary interstitium becomes hyper-osmolar.
Filtrate entering the descending limb of the LOH now passes by the hyper-osmolar medullary interstitium. Because the walls of the descending limb are water permeable, water moves down its osmotic gradient into the medullary interstitium. As water leaves, the remaining filtrate becomes more concentrated; that is, the osmolarity increases (3).
Filtrate moves along the LOH, until it reaches the thin ascending limb (4). Here, ions move out of the tubule into the interstitium down a concentration gradient. Ions continue to move into the interstitium in the thick ascending limb, but by secondary active transport (5).
This countercurrent multiplier mechanism results in a medullary interstitial osmolar gradient, with the tip of the LOH having the highest osmolarity (1200 mOsmol/L) (6).
As the LOH is highly metabolically active, it requires a good blood supply. However, if blood vessels were to simply pass through the renal medulla, they would carry solutes away, washing away the osmotic gradient (Figure 69.3a). To avoid this problem, the LOH has a specialised blood supply. The vasa recta – arteriolar branches of the efferent arterioles – follow the LOH deep into the medulla, descending with the ascending limb of the LOH, turning a hairpin bend and ascending with the descending limb to form a ‘countercurrent’ flow of blood. The hairpin design of the vasa recta is important in the maintenance of the medullary osmolar gradient (Figure 69.3b):
As the vasa recta descend in the medulla, the electrolyte content and osmolarity of their blood equilibrates with that of the surrounding interstitium: ions diffuse into the vessel and water diffuses out.
As the vasa recta ascend, their contents equilibrate with the surrounding interstitium: water diffuses into the vessel and solutes diffuse out.
Blood flows sufficiently slowly in the vasa recta to allow near-complete equilibration between the blood and the medullary interstitium. The osmolarity of the blood leaving the vasa recta is near normal (around 320 mOsmol/L). Therefore, the interstitial medullary osmolar gradient is minimally disturbed.
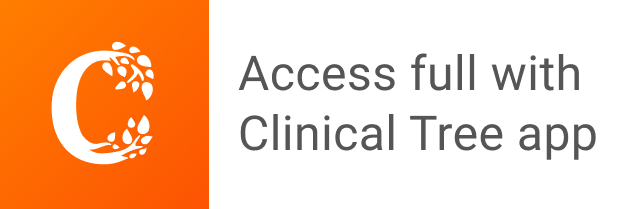