Abstract
The arterial system is the high-pressure component of the systemic circulation. The arterial system divides from the aorta into large arteries, smaller arteries and finally arterioles before joining the capillary networks. Arteries normally carry fully oxygenated blood to the capillaries. The two exceptions are the pulmonary arteries and the umbilical arteries in the foetus.
The arterial system is the high-pressure component of the systemic circulation. The arterial system divides from the aorta into large arteries, smaller arteries and finally arterioles before joining the capillary networks. Arteries normally carry fully oxygenated blood to the capillaries. The two exceptions are the pulmonary arteries and the umbilical arteries in the foetus.
Describe the cross-section of an artery
A thick muscular wall surrounds the normally circular arterial lumen. The arterial wall is made up of three layers:
Tunica externa (formerly known as the tunica adventitia), the outermost layer, made up of loose connective tissue, such as collagen fibres.
Tunica media, a thick layer of circumferential smooth muscle and elastic tissue.
Tunica intima, the innermost layer, comprising a single layer of endothelial cells. Some larger vessels also have a subendothelium, made up of connective tissue and basement membrane.
Larger arteries have their own blood supply: the vasa vasorum, a network of small blood vessels that supply the outer layers of the arterial wall.
Are there different types of artery?
Arteries are subclassified into two types:
Elastic arteries: the more proximal, larger arteries; that is, the aorta and its immediate branches. Their role is the conduction of blood. The relatively large radius of these arteries results in their resistance to blood flow being low. Elastic arteries have more elastic tissue than muscular tissue in their tunica media, allowing them to accommodate the high pressure generated by the heart during systole. The arteries expand in systole, accommodating the blood ejected from the heart, and recoil in diastole, thereby maintaining the diastolic pressure. The expansion and recoil of the elastic arteries acts to dampen the pulsatile arterial blood flow.
Muscular arteries: medium-sized arteries, which follow on from the large elastic arteries and, in turn, divide into the smaller ‘resistance’ arterioles. Muscular arteries supply individual organs; examples include the renal and coronary arteries. Their tunica media are composed primarily of smooth muscle, whose diameter is tonically controlled by the sympathetic nervous system:
– Increased sympathetic activity results in smooth muscle contraction (vasoconstriction), which narrows the vessel lumen and reduces blood flow.
– Reduced sympathetic activity permits vasodilatation, which increases the lumen diameter and increases blood flow. The mechanism for vasodilatation in this context is an increase in sheer stress cause by higher intravascular pressure. This results in a rise in intracellular Ca2+, leading to increased nitric oxide synthase activity in endothelial cells, and thus increased nitric oxide production. Nitric oxide acts as a potent vasodilator of vascular smooth muscle.
What is the mathematical relationship between vessel radius and resistance to blood flow?
Laminar flow of an incompressible Newtonian fluid of constant viscosity in a rigid tube with a circular cross-section is normally governed by the Hagen–Poiseuille equation:
Key equation: Hagen–Poiseuille equation

where ΔP is the pressure drop along the tube, Q̇ is flow, η is the viscosity, l is the tube length and r is the radius.
The equation can also be rearranged to give:

Darcy’s law states: pressure = flow × resistance; so, if ΔP is pressure and Q̇ is flow, then the resistance to flow is 8ηl/πr4.
According to the Hagen–Poiseuille equation, the most important factor affecting flow is the tube radius, owing to its fourth power. For example, doubling the radius produces a 16-fold increased flow. However, the Hagen–Poiseuille equation provides only an approximation of blood flow, because:
The pressure drop along the vessel is not continuous – it has a pulsatile component.
Blood does not behave like a Newtonian fluid. Instead, its viscosity varies with:
– Flow rate. At low blood flow, there is increased interaction between red blood cells (RBCs): they aggregate into rouleaux, thereby increasing blood viscosity, and thus resistance to flow is greater. At normal flow rates, aggregation is prevented by negatively charged sialic acid residues on the RBC surface repelling other RBCs.
– Haematocrit. Interactions between RBCs mean that high haematocrit is associated with a greater overall blood viscosity. In anaemia, a reduced haematocrit increases blood flow; a haematocrit of around 0.3 is considered to be the optimal balance between blood flow and O2-carrying capacity.
– Temperature. Viscosity increases as temperature decreases. This has some relevance in intensive care: until recently, patients were cooled following cardiac arrest and therefore had an increased blood viscosity. Thus, blood flow was decreased at a time when flow may already be low due to coexisting cardiogenic shock.
– Fahraeus–Lindqvist effect. At vessel diameters below 300 μm (i.e. arterioles), RBCs tend to stream towards the centre of the vessel, leaving the plasma at the vessel walls. As plasma has a lower viscosity than whole blood, the resistance to blood flow is reduced. This Fahraeus–Lindqvist effect opposes the tendency for resistance to increase as the vessel radius decreases, especially in arterioles and capillaries.
Vessels are not uniform rigid tubes.
– Vessels are non-uniform. They may have branches, turn corners or be narrowed due to atherosclerotic plaques or external compression. These factors increase the risk of turbulence.
– Vessels are distensible. According to the Hagen–Poiseuille equation, a rigid tube has a constant resistance: flow is therefore proportional to pressure (Figure 34.1). However, large vessels are distensible: an increase in pressure causes an increase in vessel radius, which reduces resistance and thus increases flow. In contrast, if the pressure falls near to zero (as may occur in veins), the vessel tends to collapse, resulting in no flow.
– Myogenic autoregulation is the intrinsic ability of an arteriole to maintain a constant blood flow despite changes in intraluminal pressure (see below) (Figure 34.1).
What are the functions of the arterioles?
The arterioles are the vessels with the smallest radii of the arterial tree and are therefore the main source of resistance to blood flow. Structurally, their tunica media is made up of one or two layers of circumferential smooth muscle. Vasoconstriction increases the vessel’s resistance to blood flow; this is very sensitive owing to the fourth-power relationship of the radius in the Hagen–Poiseuille equation. Likewise, vasodilatation reduces the vessel’s resistance to blood flow. Compared with arteries, the arterioles lack elastin in their tunica media. Pulsatile blood flow is damped, becoming continuous flow by the time the blood reaches the capillaries.
In addition to conducting blood from small arteries to the capillaries, the arterioles have three other roles:
Control of the distribution of blood flow to different organs by altering organ vascular resistance;
Control of total systemic vascular resistance (SVR);
Alteration of capillary hydrostatic pressure, effectively controlling bulk flow of water between intravascular and interstitial body fluid compartments (see Chapter 36).
Which factors are involved in arteriolar vasoconstriction and vasodilatation?
The control of arteriolar smooth muscle is complex, influenced by local, humoral and neural factors.
Local factors:
– Myogenic autoregulation. This is an intrinsic property of the arteriolar smooth muscle in which the vessel vasoconstricts in response to increased intraluminal pressure. This occurs because mechanically gated ion channels in the vascular wall are opened by stretch, leading to the opening of voltage-gated Ca2+ channels, Ca2+ influx and thus to contraction of arteriolar smooth muscle. Likewise, arterioles vasodilate in response to reduced intraluminal pressure via the sheer stress mechanism. The overall effect is the maintenance of a relatively constant blood flow over a range of intraluminal pressures. Autoregulation is an important feature of arterioles in the brain, heart and kidney, but does not occur in the skin.
– Metabolites. The tissues regulate blood flow in proportion to their metabolic rate. When an organ increases its metabolic activity, the local O2 tension falls and the concentration of metabolites (CO2, H+ ions, lactate) increases. The arteriole vasodilates in response to increased metabolite concentration, increasing blood flow and therefore O2 delivery to the tissues. This is thought to be the mechanism behind reactive hyperaemia: the large increase in blood flow following temporary cessation of organ perfusion (e.g. critical limb ischaemia) or following deflation of an arterial tourniquet in limb surgery.
Humoral factors. A number of locally and systemically produced chemical substances affect arteriolar smooth muscle tone, including:
– Kinins (e.g. bradykinin), which cause vasodilatation in the salivary glands, gut and skin.
– Histamine, released from basophils and mast cells as part of the inflammatory response. The resulting arteriolar vasodilatation increases blood flow to the affected tissues. During allergic reactions, the systemic release of histamine is responsible for widespread arteriolar vasodilatation.
– Nitric oxide (NO), previously known as endothelium-derived relaxing factor, released from the endothelium in response to shear stress. NO is a potent arteriolar vasodilator, increasing blood flow to the damaged area. NO also causes venodilatation; this is the mechanism underlying a technique familiar to all anaesthetists: tapping the skin overlying a peripheral vein prior to cannulation.
– Serotonin and thromboxane A2 are released from platelets when they are activated (e.g. when a blood vessel is cut). Both chemicals cause arteriolar vasoconstriction, which reduces the local blood flow, allowing the clot to form without it being washed away.
– Adrenaline activates both α1-adrenoceptors, resulting in vasoconstriction, and β2-adrenoceptors, resulting in vasodilatation. The response of arterioles to adrenaline differs between organs depending on their relative proportions of α1– and β2-adrenoceptors. In the gut and skin, there is a high concentration of arteriolar α1-adrenoceptors: adrenaline causes vasoconstriction, resulting in reduced blood flow. In contrast, heart and skeletal muscles have high concentrations of β2-adrenoceptors, the activation of which causes vasodilatation.
– Anti-diuretic hormone (ADH; or arginine vasopressin), which is synthesised in the hypothalamus and released from the posterior lobe of the pituitary gland in response to low plasma volume and high plasma osmolarity. In addition to its anti-diuretic effect on the collecting ducts, ADH is a potent arteriolar vasoconstrictor. This effect is unimportant in health, but it is an important mechanism for maintaining blood pressure in haemorrhage. Vasopressin is used clinically as an arteriolar vasoconstrictor in the intensive care unit: patients with septic shock have been shown to have relatively reduced ADH concentrations.
– Other hormones. Angiotensin II is a potent arteriolar vasoconstrictor, whilst atrial natriuretic peptide is an arteriolar vasodilator that also decreases the sensitivity of the arterioles to other vasoconstrictors. Together with ADH, these hormones are normally concerned with control of blood volume and total body water.
Neural factors:
– Sympathetic nervous system. The arterioles have a rich sympathetic nervous supply; the basal sympathetic outflow is responsible for setting the resting arteriolar smooth muscle tone. Activation of the sympathetic nervous system results in the release of noradrenaline from post-ganglionic neurons, which, like adrenaline, activates arteriolar α1-adrenoceptors, resulting in vasoconstriction. In contrast to adrenaline, noradrenaline only weakly activates β2-adrenoceptors, causing minimal arteriolar vasodilatation. Sympathetic nervous stimulation causes arteriolar vasoconstriction in the skin, kidneys and gut, where α1-adrenoceptors are plentiful, but has minimal effect on the arterioles of the brain and heart, which have relatively few α1-adrenoceptors. Sympathetic stimulation therefore preferentially directs blood flow to these vital organs.
– Parasympathetic nervous system. Parasympathetic control of arteriolar smooth muscle tone is only found in the penis, where parasympathetic stimulation results in vasodilatation and erection.
– Skeletal muscle. Skeletal muscle is unusual1 in that it possesses a sympathetic cholinergic pathway, which is only activated following the onset or anticipation of exercise, fear, anger or pain. ACh is released at post-ganglionic nerve endings instead of noradrenaline, resulting in arteriolar vasodilatation and a substantial increase in skeletal muscle blood flow.
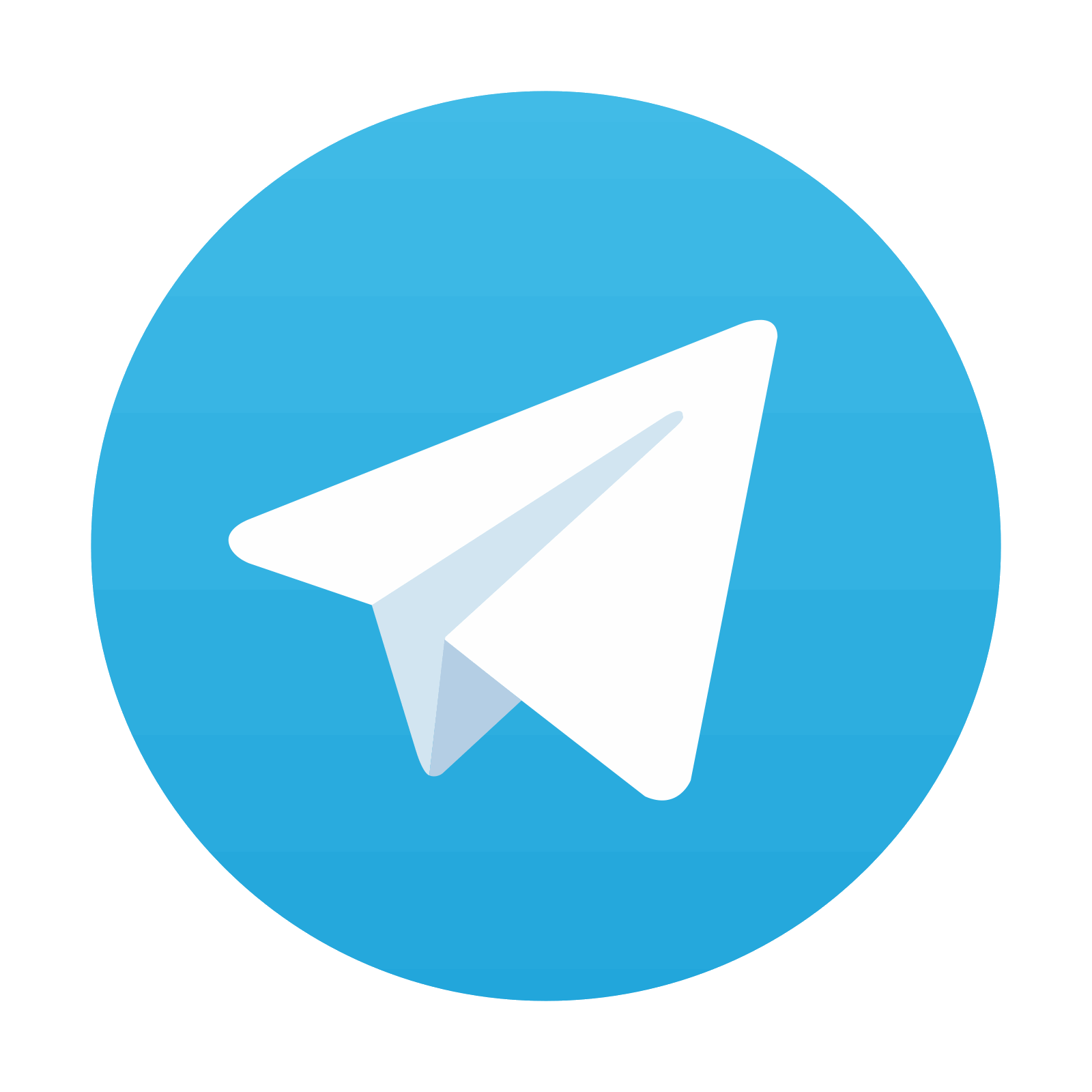
Stay updated, free articles. Join our Telegram channel
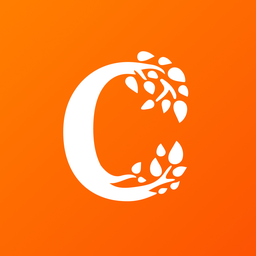
Full access? Get Clinical Tree
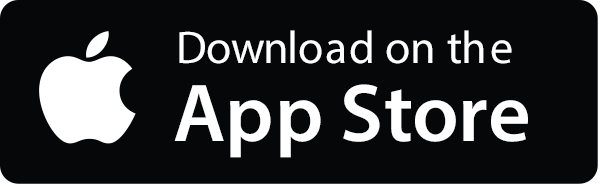
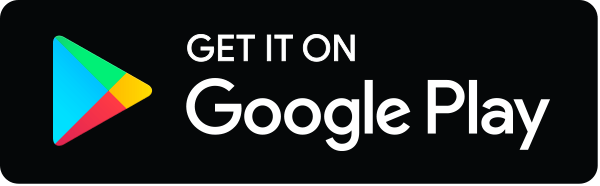