Abstract
The metabolic demands of the brain are substantial and must be met with a continuous supply of glucose and oxygen. The cerebrovascular anatomy and physiology is designed to distribute and regulate the flow of blood throughout the cranium to continuously meet these demands. Distribution occurs through a well-developed system of conducting vessels that form a proximal anastomotic loop referred to as the circle of Willis. Adjacent vascular territories also communicate via small leptomeningeal collaterals on the surface of the brain. Cerebral blood flow is regulated and influenced by chemical mediators, myogenic regulation, neurogenic input, blood viscosity, patient age, vasoactive agents, anesthetics, and various CNS injury states. Neuronal dysfunction occurs when CBF is reduced below 20 mL/100 g/min. Further reductions below 15 mL/100 g/min result in a progressive decrease in energy supply that will lead to membrane failure and death over the time course of hours to minutes depending of the level of CBF. Energy failure is characterized by cell membrane depolarization, influx of Na + , and Ca 2+ , and downstream cell signaling pathways that result in cell damage and death. Ischemia induced cell death may be apoptotic or necrotic depending on various factors involved. More recent understanding of ischemic injury suggests that it is a dynamic process that continues well beyond the initial ischemic event. While anesthetic agents can reduce the vulnerability of the brain to ischemic injury, maintenance of physiologic homeostasis is of greater importance in the prevention or exacerbation of ischemic injury.
Keywords
cerebral blood flow, chemical regulation, flow metabolism coupling, myogenic regulation, neurogenic regulation, volatile anesthetics, intravenous anesthetics
Chapter Outline
Regulation of Cerebral Blood Flow
Chemical Regulation of Cerebral Blood Flow
Myogenic Regulation (Autoregulation) of Cerebral Blood Flow
Neurogenic Regulation of Cerebral Blood Flow
Viscosity Effects on Cerebral Blood Flow
Cerebral Spinal Fluid Dynamics
Pathophysiology of Cerebral Ischemia
This chapter focuses on central nervous system (CNS) physiology and pharmacology relevant to anesthesiology. The molecular and cellular substrates of specific drugs affecting the nervous system are covered in Chapter 8 and in the appropriate chapters in this section.
Cerebrovascular Anatomy
The cerebral arterial vasculature is divided into anterior and posterior circulations that are interconnected through a vascular loop referred to as the circle of Willis . This anastomotic loop provides potential for collateral blood flow between the anterior and posterior circulations, as well as between cerebral hemispheres in case of impaired flow in one of the proximal feeding vessels. As one of the most highly perfused organs in the body, the brain is supplied by two paired arteries: the right and left vertebral and the right and left internal carotid arteries. Flow is then distributed throughout the cranium primarily by three paired cerebral arteries arising from the circle of Willis: the anterior cerebral, middle cerebral, and posterior cerebral arteries ( Figs. 9.1 and 9.2 ). However, a complete circle of Willis may be present in only about 40% to 50% of the population. A variety of incomplete (containing a noncontinuous, hypoplastic, or absent segment) variants that can compromise collateral flow have been identified. In such cases, secondary sources of collateral flow can occur through distal leptomeningeal connections, or by way of retrograde flow though the ophthalmic artery from the external carotid circulation to the internal carotid artery.



Vascular Architecture
Arteries and Arterioles
The cerebral arteries distributing flow from the circle of Willis continuously branch into smaller arteries and arterioles that course along the surface of the brain. At their distal end, before entering the brain parenchyma, they are known as leptomeningeal vessels. The leptomeningeal vessels are found within the pia-arachnoid membrane and are surrounded by cerebral spinal fluid (CSF). The highly branched pial arterioles form an extensive collateral network on the surface of the brain before giving rise to a relatively unbranched system of penetrating intraparenchymal arterioles.
Venous Circulation
The venous circulation consists of an interconnected system of veins and sinuses. Venous outflow from the cerebral hemispheres occurs via cortical veins within the pia mater on the surface of the brain as well as deep central veins. Cortical and deep veins may empty into the superior sagittal sinus. Deep veins may also empty into either the inferior sagittal sinus or great vein of Galen. Venous flow is directed toward a confluence of sinuses (torcular herophili) then on toward the central circulation via the transverse sinus, sigmoid sinus, and jugular vein ( Fig. 9.3 ). Cerebellar drainage occurs primarily via the inferior cerebellar veins and occipital sinuses. Extensive collateralization between cortical veins as well as the deep veins and sinuses can augment venous drainage when primary routes are compromised.

Anterior Circulation
The anterior circulation arises from the intracranial extension of the internal carotid arteries. The most prominent branches of the anterior circulation are the anterior cerebral, middle cerebral, and posterior communicating (PCom) arteries. The PCom provides communication with the posterior circulation through direct connection with the posterior cerebral artery (PCA). An absent or hypoplastic PCom potentially compromises collateral flow between the anterior and posterior circulations. Likewise, collateral flow between the left and right anterior circulation can be compromised by a hypoplastic or aplastic anterior communicating (ACom) artery.
Posterior Circulation
The posterior circulation arises from paired vertebral arteries. At their rostral end, the vertebral arteries typically join together on the anterior surface of the brainstem to form the basilar artery. The vertebral and basilar arteries have multiple branches that supply the thalami, brainstem, cerebellum, and spinal cord. The basilar artery branches distally into the left and right PCAs. The branches of the PCA provide blood flow to the thalami, brainstem, choroid plexus, and cerebrum (occipital and portions of parietal and temporal lobes see Fig. 9.2A, B ) and provide a point of connection with the anterior circulation. Like the anterior circulation, there are many anatomic variants that may compromise collateral blood flow and predispose to ischemia in various clinical scenarios.
Regulation of Cerebral Blood Flow
The adult brain represents about 2% of total body weight (1350 g), but receives almost 15% of cardiac output. This high blood flow:mass ratio reflects the significant metabolic demand of the brain. At rest, it consumes oxygen at an average rate of 3.5 mL/100 g of brain tissue per minute, or 50 mL/min overall. This represents nearly 20% of total body oxygen utilization. Normal values for cerebral blood flow (CBF), cerebral metabolic rate (CMR), and other physiologic variables are provided in Table 9.1 .
Cerebral Blood Flow | |
Global | 45–55 mL/100 g per minute |
Cortical (mostly gray matter) | 75–80 mL/100 g per minute |
Subcortical (mostly white matter) | 20 mL/100 g per minute |
Cerebral metabolic rate of oxygen (CMRO 2 ) | 3–3.5 mL/100 g per minute |
Cerebral venous P o 2 | 32–44 mm Hg |
Cerebral venous S o 2 | 55%–70% |
Intracranial pressure (supine) | 10–15 mm Hg |
Approximately 60% of cerebral energy consumption is used to support electrophysiologic function in the form of maintenance and restoration of ionic gradients as well as the synthesis, transport, and reuptake of neurotransmitters. This functional component of cerebral energy consumption is reflected as electrical activity on electroencephalography (EEG) and is reduced by most anesthetic drugs. Maintenance of cellular homeostasis, which includes synthesis and transport of cellular components, accounts for the remainder of oxygen consumption. This basal component of neuronal energy consumption related to basic cellular housekeeping function is independent of electrophysiologic activity but is responsive to temperature manipulation.
Glucose is the primary substrate of brain metabolism. In the presence of adequate oxygen delivery, glucose is metabolized to pyruvate by oxidative phosphorylation. In the absence of sufficient oxygen delivery, glucose is anaerobically metabolized, resulting in insufficient adenosine triphosphate (ATP) production and subsequent energy failure. Therefore the brain’s considerable metabolic demands must be met by adequate and continuous delivery of both oxygen and glucose. There are elaborate mechanisms in place for regulating CBF to ensure these demands are met. The various mechanisms, which include chemical, myogenic, and neurogenic factors, are listed in Table 9.2 .
Chemical/Metabolic/Humoral |
|
Myogenic |
Autoregulation b |
Rheologic |
Blood viscosity |
Neurogenic c |
|
a Cerebal metabolic rate influence assumes intact flow-metabolism coupling.
b The autoregulation mechanism is fragile, and in many pathologic states cerebral blood flow is regionally pressure-passive.
c The contribution and clinical significance is poorly defined.
Chemical Regulation of Cerebral Blood Flow
A number of factors, by altering the biochemical milieu of the brain, modulate CBF; these include CMR, partial pressure of carbon dioxide in arterial blood (Pa co 2 ), and alveolar oxygen partial pressure (Pa o 2 ).
Cerebral Metabolic Rate
CMR is directly proportional to neuronal activity. Although the mechanism is not entirely understood, an increase in neuronal activity and CMR is tightly coupled to increased CBF. This physiologic process, wherein blood flow and metabolism are matched, is referred to as flow-metabolism coupling. The cellular mechanisms involved continue to be investigated, but likely involve processes mediated by a combination of neuronal activity and metabolic by-products, glial integration and modulation of neuronal synaptic activity, and vascular factors. Specific by-products of metabolism considered influential in this process include potassium ions (K + ), hydrogen ions (H + ), calcium ions (Ca 2+ ), lactate, adenosine, ATP, and nitric oxide (NO). Glia are in direct contact with both neurons and capillaries, and mounting data highlight the role of glia as a likely conduit for coupling neuronal activity with regional blood flow ( Fig. 9.4 ). Cerebral vessels are also densely innervated, and peptidergic neurotransmitters released by nerves also contribute to vascular regulation and flow-metabolism coupling. In the neurosurgical and critical care environments, CMR is influenced by the functional state of the nervous system, anesthetic agents, and temperature.

Functional State
CMR decreases during sleep and increases during sensory stimulation, mental tasks, or arousal of any cause. CMR is markedly increased during epileptic activity and substantially reduced in coma.
Anesthetic Agents
In general, with the exception of ketamine and nitrous oxide (N 2 O), anesthetics suppress CMR. Specifically, the component of CMR related to electrophysiologic function is reduced. With several agents, including barbiturates, isoflurane, sevoflurane, desflurane, propofol, and etomidate, increasing plasma concentrations cause progressive suppression of EEG activity with a concomitant reduction in CMR. However, increasing the plasma level beyond that required to achieve suppression of the EEG results in no further CMR reduction. The component of CMR related to basic cellular homeostatic activities is unaffected by anesthetics ( Fig. 9.5 ).

Temperature
CMR decreases by 6% to 7% per degree Celsius of temperature reduction, and can cause complete suppression of the EEG at about 18°C to 20°C. In contrast to anesthetic agents, temperature reduction beyond that at which EEG suppression first occurs does produce a further decrease in CMR. This occurs because hypothermia decreases the rate of energy utilization associated with both electrophysiologic function and basal maintenance of cellular integrity ( Fig. 9.6 ). These decreases were once assumed to be proportional, but subsequent data demonstrate preferential suppression of the basal component (with mild hypothermia). The global cerebral metabolic rate of oxygen (CMRO 2 ) at 18°C is less than 10% of normothermic control values, which likely accounts for the brain’s tolerance of circulatory arrest for moderate periods at these temperatures.

Hyperthermia leads to substantial increases in CMR and CBF. However, above 42°C a dramatic reduction in CMRO 2 occurs, possibly owing to neuronal injury and protein denaturation.
Paco 2
CBF varies directly with Pa co 2 ( Fig. 9.7 ). For each change of 1 mm Hg in Pa co 2 from normal values, CBF changes 1 to 2 mL/100 g per minute. This response is attenuated outside the physiologic range of about 20 to 80 mm Hg and is sensitive to the baseline CBF. For example, hyperventilation causes a greater reduction in CBF when the resting CBF is high (as can occur with volatile anesthetics), an effect that is attenuated with lower resting CBF. Therefore anesthetic agents have the capacity to alter CO 2 responsiveness of the cerebral circulation by changing basal CBF.

The changes in CBF caused by Pa co 2 depend on the pH in the extracellular fluid of the brain. Extracellular pH and CBF changes occur rapidly after Pa co 2 adjustments because CO 2 (neutral charge) diffuses freely across the cerebrovascular endothelium. In contrast, acute systemic metabolic acidosis has little immediate effect on CBF because the blood-brain barrier (BBB) excludes H + from the perivascular space.
Although changes to CBF induced by Pa co 2 are rapid, they are not sustained. Despite maintenance of increased arterial pH (through hyperventilation), CBF returns toward baseline over 6 to 18 hours because CSF pH gradually normalizes through bicarbonate extrusion ( Fig. 9.8 ). Therefore patients with sustained periods of hyperventilation or hypoventilation deserve special consideration. Acute normalization of Pa co 2 results in a significant CSF acidosis (after hypocapnia) or alkalosis (after hypercapnia). The former results in increased CBF with a concomitant increase in intracranial pressure (ICP), depending on the prevailing intracranial elastance. The latter conveys the theoretical risk of ischemia.

Pao 2
The effects of Pa o 2 on CBF are minimal within the normal physiologic range (Pa o 2 from 60 to more than 300 mm Hg). Below a Pa o 2 of 60 mm Hg, CBF increases rapidly (see Fig. 9.7 ). The mechanisms mediating cerebral vasodilation during hypoxemia remain elusive, but they appear to be influenced by metabolites produced by nonvascular cerebral tissue (e.g., NO), hypoxic effects directly influencing cerebral vascular endothelium and smooth muscle (e.g., hypoxia-induced hyperpolarization of smooth muscle), and stimulation of the receptors in the rostral ventrolateral medulla. The response to hypoxemia is synergistic with hypercarbia-induced hyperemia.
Myogenic Regulation (Autoregulation) of Cerebral Blood Flow
Autoregulation refers to the capacity of the cerebral circulation to alter vascular resistance to maintain a relatively constant CBF over a range of mean arterial pressure (MAP). Although animal models suggest a lower limit of autoregulation (LLA) near a MAP of 50 mm Hg, available human data suggest a higher threshold (with considerable interindividual variation). In normal nonanesthetized humans, the limits of autoregulation occur near MAPs of approximately 70 and 150 mm Hg (see Fig. 9.7 ). These limits are shifted toward the right in patients with poorly controlled, longstanding hypertension. Note that the units used on the x -axis of “autoregulation curves” will influence the correct inflection points of the curve. When the x -axis is MAP, the normal average LLA is near 70 mm Hg. Cerebral perfusion pressure (CPP) is the ideal independent variable. However, ICP is usually not measured in normal subjects, so CPP (MAP-ICP) is rarely available. Assuming a normal ICP in a supine subject of 10 to 15 mm Hg, an LLA of 70 mm Hg MAP corresponds to 55 to 60 mm Hg expressed as CPP. Above and below the autoregulatory limits, CBF becomes pressure-dependent (pressure-passive) and varies linearly with CPP. The autoregulatory response to a change in arterial pressure occurs over the course of 1 to 2 minutes, such that a rapid change in arterial pressure will transiently alter CBF even within the normal autoregulatory range.
The mechanisms governing autoregulation are unclear. According to the myogenic hypothesis, changes in CPP directly influence alterations in vascular smooth muscle tone independent of extrinsic factors. However, autonomic innervation of cerebral blood vessels might also contribute to autoregulation (discussed in later text).
Autoregulatory mechanisms may be absent or significantly attenuated in pathologic conditions, such as cerebral ischemia, traumatic brain injury, and subarachnoid hemorrhage ( Fig. 9.9 ). In addition, volatile anesthetics dose dependently attenuate autoregulation, an effect attributable to direct vasodilation.
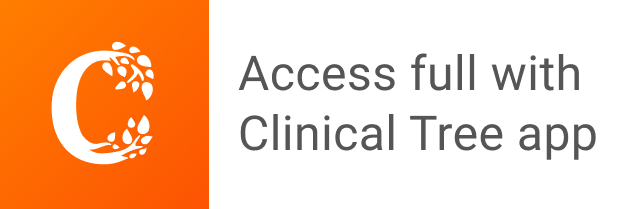