












characterized by the rapid expression of a unique group of proteins known collectively as heat shock proteins (HSPs), when a cell or organism is exposed to environmental stress. The structure, mode of regulation, and function of HSPs are highly conserved among different species, and HSPs have been identified in virtually all eukaryotic and prokaryotic species examined. While classically described as a response to heat stress (hence the term heat shock response), HSPs can be induced by a wide variety of nonthermal stressors and pharmacologic agents (Table 20.1). For this reason, the terms “stress response” and “stress proteins” may be descriptors that are more appropriate. Many of these stimuli are relevant to the critically ill patient, and it is now well established that critically ill patients readily express HSPs (i.e., mounting a heat shock response) (4,5,6,7). Moreover, and, particularly germane to pediatric critical care medicine, the experimental literature suggests that younger animals have a greater capacity to express HSPs than older animals (8). Indeed, the loss of the ability to generate a stress response may contribute to the aging process (9,10).
(discussed in detail later in this chapter). In addition, ubiquitin, inhibitor of κB (IκB), endothelial nitric oxide synthase (eNOS), and mitogen-activated protein kinase phosphatase-1 (MKP-1) are listed as HSPs in that they can be induced by classic heat shock. Many HSPs are constitutively expressed (e.g., HSP90 is one of the most abundant proteins found inside the cell), while others are rapidly and highly expressed in response to cellular stress. Among the latter, HSP72 is one of the more well-studied inducible HSPs in the context of cellular adaptations to stress and consequent protection against cellular injury (see in what follows).
TABLE 20.1 INDUCERS OF THE STRESS RESPONSE | ||||||||||
---|---|---|---|---|---|---|---|---|---|---|
|
TABLE 20.2 THE MAJOR FAMILIES OF HEAT SHOCK PROTEINS | ||||||||||||||||||||||||||||||||||||||||||||||||||
---|---|---|---|---|---|---|---|---|---|---|---|---|---|---|---|---|---|---|---|---|---|---|---|---|---|---|---|---|---|---|---|---|---|---|---|---|---|---|---|---|---|---|---|---|---|---|---|---|---|---|
|

inflammation (see Chapter 19). Heat shock-mediated inhibition of the NF-κB pathway involves inhibition of IκB kinase and increased de novo expression of the endogenous NF-κB inhibitory protein, IκBα. These inhibitory effects of heat shock on cellular proinflammatory responses and the NF-κB pathway appear to be relatively specific, rather than a global downregulation of cellular function and gene expression. Furthermore, genome-level studies indicate that the mononuclear cell response to heat stress is highly divergent compared with the mononuclear cell response to endotoxin (15).

![]() FIGURE 20.2. HO-mediated degradation of heme. HO degrades heme to biliverdin and concomitantly generates CO and iron (Fe). Biliverdin is subsequently converted to bilirubin via biliverdin reductase. The respective colorimetric reactions that coincide with the generation of these heme degradation products are readily evident in the various hues of a common bruise. |
For example, adult patients suffering from major trauma have increased serum levels of HSP72 (4). Critically ill children with septic shock also have increased serum levels of HSP72, and the absolute levels are much higher than that reported for critically ill adult patients following multisystem trauma (5). The latter may be reflective of an increased capacity of children to express HSPs compared with adults, consistent with the experimental literature previously mentioned.

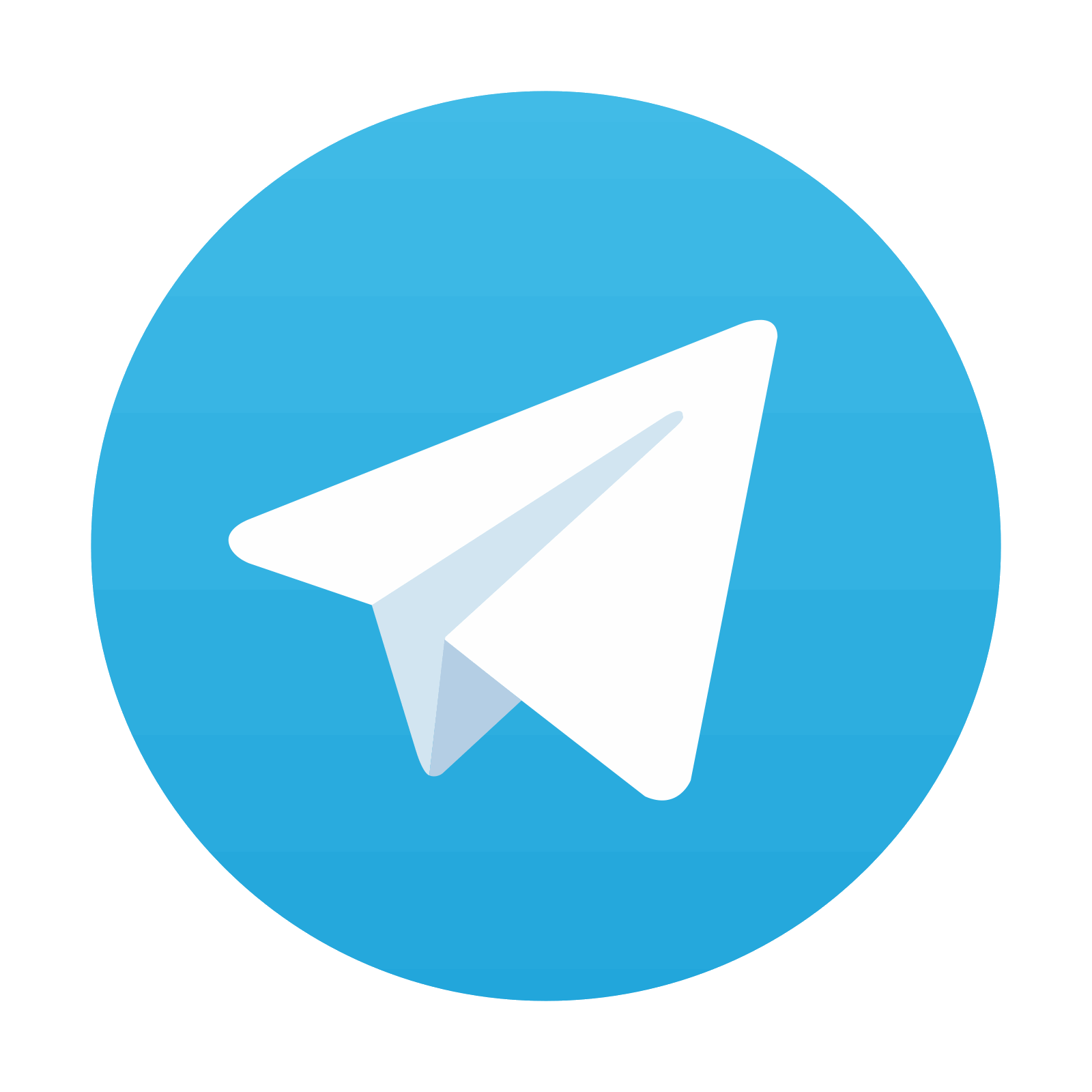
Stay updated, free articles. Join our Telegram channel
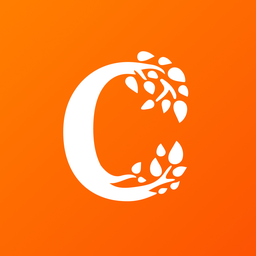
Full access? Get Clinical Tree
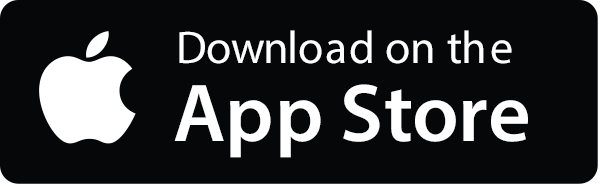
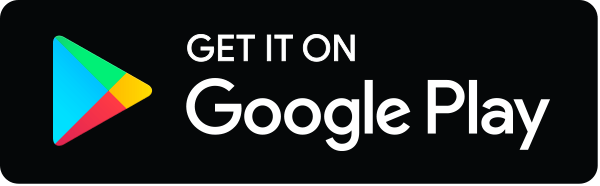
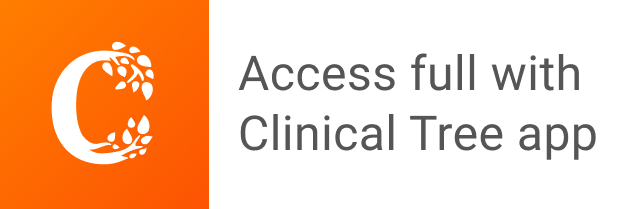