Chapter 23
Cardiovascular Anatomy, Physiology, Pathophysiology, and Anesthesia Management
Cardiovascular System
Heart
The heart is bound anteriorly by the sternum and the costal cartilages of the third, fourth, and fifth ribs and inferiorly by the diaphragm. It is positioned with the apex of the heart projecting anteriorly and inferiorly toward the left fifth intercostal space at the midclavicular line. At this location, the pulsation from the cardiac apex may be palpated. This is known as the point of maximal impulse. The first heart sound (S1) is best auscultated in this area. A third (S3) or fourth (S4) heart sound, if present, can also be heard in this location. Heart sounds are generated from the vibrations caused by the closure of the semilunar and atrioventricular (AV) valves.1
Pericardium
The heart is situated within the mediastinum and surrounded by a fibrous, double-walled sac called the pericardium,2 which envelops the heart and the roots of the great vessels. It consists of a visceral portion, which is in intimate contact with the outer surface of the heart (epicardium), and an outer parietal portion, and adheres to the fibrous pericardium (Figure 23-1).
The fibrous pericardium is pierced superiorly by the aorta, the pulmonary trunk, and the superior vena cava. The base of the fibrous pericardium is fused with the central tendon of the diaphragm. The visceral pericardium and parietal pericardium are separated by a thin potential space known as the pericardial cavity. This space normally contains approximately 10 to 25 mL of serous fluid, which provides lubrication for the free movement of the heart within the mediastinum. In disease states, the pericardial space can fill with blood and or serosanguinous fluid, compress the heart, and decrease cardiac output (CO). In acute cardiac tamponade, the volume rapidly increases, producing myocardial dysfunction. In contrast, in chronic cardiac tamponade, the degree of pressure exerted on the heart increases slowly because the pericardial sac stretches over time to accommodate the blood that accumulates. However, the pressure may eventually increase as much as 10-fold before symptoms of cardiac tamponade occur.3
Surface Anatomy
The atria are separated from the ventricles by the coronary sulcus (AV sulcus), as seen in Figure 23-2, A. The right coronary artery travels within this sulcus. The circumflex artery arises from the left coronary artery and travels in the coronary sulcus until it branches posteriorly. The RV and LV are separated by the interventricular sulci, which descend from the coronary sulcus to the apex. The interventricular sulci are composed of an anterior interventricular sulcus and a posterior interventricular sulcus. The anterior interventricular sulcus contains the left anterior descending (LAD) artery, which courses over the interventricular septum and continues in the posterior interventricular sulcus.
The crux of the heart is the place at which the coronary and the posterior interventricular sulci meet. Internally, it is where the atrial and ventricular septa meet (Figure 23-2, B). This anatomic crux is important in determining coronary artery dominance.
Cardiac Skeleton
Essential to a discussion of the chambers of the heart is a description of the fibrous skeleton, the annulus fibrosus (Figure 23-3). Tough fibrous rings surround the AV valves and act as points of attachment for the valves. Two additional fibrous annuli develop in relation to the bases of the aorta and the pulmonary trunk. The aortic fibrous annulus is connected to the pulmonary annulus by a fibrous band called the tendon of the conus. The aortic annulus is connected to the AV annuli by the small left fibrous trigone and the larger right fibrous trigone, also called the central fibrous body. The four annuli and their interconnections constitute the fibrous cardiac skeleton.
Chambers of the Heart
Right Atrium
The RA receives blood from several sources: the superior vena cava, the inferior vena cava, and the coronary sinus (Figure 23-4). The RA consists of two parts: an anterior, thin-walled trabeculated portion and a posterior, smooth-walled portion called the sinus venarum. The sinus venarum receives blood from the vena cava and the coronary sinus. The auricle projects to the left from the root of the superior vena cava and overlaps the root of the ascending aorta.
The superior vena cava returns blood to the RA from the upper body. The inferior vena cava returns blood to the RA from the lower body. The entrance of the inferior vena cava into the RA is protected by a rudimentary valve called the eustachian valve.4
The entrance from the coronary sinus into the RA is located between the AV orifice and the valve of the inferior vena cava. This opening is protected in part by a rudimentary valve of the coronary sinus called the thebesian valve.5 Other distinguishing structures in the RA include the interatrial septum and the fossa ovalis cordis, which is the remnant of the fetal foramen ovale within the septum.
Right Ventricle
The RV ejects blood into the pulmonary arterial system for oxygenation and removal of carbon dioxide by the lungs. The RV communicates with the RA through the AV orifice, which is separated by the tricuspid valve. The RV also communicates with the pulmonary outflow tract through the pulmonary orifice, which is guarded by the pulmonic valve (see Figure 23-4).
The walls of the RV are much thicker (4 to 5 mm) than those of the RA because of the increased pressures required to generate forward blood flow into the pulmonary circulation. The superior portion of the RV as it approaches the pulmonary orifice has a conical appearance and is called the conus arteriosus or infundibulum.6
The inner wall of the conus is smooth, but the remainder of the right ventricular wall has a rough appearance because of the presence of several irregular muscular bundles called the papillary muscles and the trabeculae carneae. One of the trabeculae carneae (the moderator band) crosses the cavity of the ventricles and carries the right branch of the AV bundle. The papillary muscles have attachments to the ventricular walls and to the chordae tendineae. The chordae tendineae are attached to the cusps of the tricuspid valve; together with the papillary muscles, they help prevent eversion of the tricuspid valve into the RA during ventricular systole.7
Valves
One of the most accurate ways to determine the presence of valvular pathology is by calculating valve area. The standard method for determining valve area is by cardiac catheterization. A cardiologist is able to determine valve gradients using the Gorlin formula or its correction, which can provide information regarding the degree of pathology that exists.9
Where valve area is expressed in cm2, blood flow across the mitral valve is expressed in mL/s, K is a hydraulic pressure constant, and mean transvalvular gradient is expressed in mmHg.
Atrioventricular Valves
Tricuspid Valve: The tricuspid valve is situated within the right AV orifice, which lies between the RA and the RV. The tricuspid leaflets are thinner and more translucent than the mitral valve and more easily separated into well-defined leaflets. Three leaflets of unequal size exist: the anterior, septal, and posterior leaflets. The leaflets are attached to the chordae tendineae, which are attached to the papillary muscles.10 The normal tricuspid valve area is approximately 7 cm2. Symptoms associated with tricuspid valve stenosis occur when the valve area is less than 1.5 cm2.
Mitral Valve: The mitral valve is situated in the left AV orifice between the LA and the LV. Two major leaflets, the anteromedial leaflet and the posterolateral leaflet, are connected by commissural tissue. The normal mitral valve area is 4 to 6 cm2. When the surface area of the valve is decreased by half, clinical symptoms may appear. Like the tricuspid valve, the mitral valve has papillary muscles and chordae tendineae attached to the leaflets to prevent eversion of the valve during ventricular systole.11
Semilunar Valves
The configuration of the aortic and pulmonary valves is similar. The cusps of the aortic valve are slightly thicker because it is subjected to greater pressures, which are created by left ventricular ejection. The semilunar valves are situated within the outflow tracts of their corresponding ventricles. Each valve is composed of three cusps. Above the aortic valve is a dilation known as the sinus of Valsalva, which allows the valve to open efficiently without occluding the coronary ostia or openings that communicate with the coronary arteries. Eddy currents form behind the valve leaflets and prevent contact between the valve leaflets and the walls of the aorta. Normal aortic valve area is 2.5 to 3.5 cm2. Reduction of the valve area by one third to one half is associated with an increase in the symptoms caused by aortic stenosis.
Coronary Circulation
Coronary Arteries
The coronary ostia are the entrance points by which blood flows through the coronary circulation and they are located behind the aortic cusps near the superior part of the sinus of Valsalva. The ostium of the left coronary artery is superior and posterior to the right coronary ostium. The coronary arteries act as end arteries, and each supplies blood to its respective capillary bed12 (Figure 23-5).
Left Main Coronary Artery: The left main coronary artery travels anteriorly, inferiorly, and leftward from the left coronary sinus to emerge from behind the pulmonary trunk. Within 2 to 10 mm of its emergence, the left main coronary artery divides into two or more branches of near-equal diameter. The branches include the LAD artery, the left circumflex coronary artery, and possibly the diagonal branch.
Left Anterior Descending Coronary Artery: The LAD is a continuation of the left main coronary artery. The branches of this vessel include the first diagonal branch, the first septal perforator, the right ventricular branches (not always observed), other septal perforators, and other diagonal branches. The LAD provides blood flow to the anterior two thirds of the interventricular septum, the right and left bundle branches, the anterior and posterior papillary muscles of the mitral valve, and the anterior lateral and apical walls of the LV. The LAD also provides collateral circulation to the anterior wall of the RV.
Left Circumflex Coronary Artery: The left circumflex artery arises from the left main coronary artery at an obtuse angle, and it is directed posteriorly as it travels around the left side of the heart within the left AV sulcus. Branches are variable and may include the sinus node artery (40% to 50% of the population), the left atrial circumflex artery, the anterolateral marginal artery, the distal circumflex artery, one or more posterolateral marginal arteries, and the posterior descending artery (10% to 15% of the population). The circumflex artery supplies blood to the left atrial wall, the posterior and lateral LV, the anterolateral papillary muscle, the AV node in 10% of the population, and the sinoatrial (SA) node in 40% to 45% of the population.
Right Coronary Artery: The right coronary artery supplies blood to the SA and AV nodes, the RA and RV, the posterior third of the interventricular septum, the posterior fascicle of the left bundle branch, and the interatrial septum. In approximately 90% of the population, the right coronary artery leaves the right coronary sinus and descends in the right AV groove. At the crux, the right coronary artery courses inferiorly in the posterior AV groove and terminates as a left ventricular branch.
Coronary Artery Dominance: Dominance of one coronary artery is determined by the location of the coronary artery that crosses the crux and provides blood flow to the posterior descending artery. The dominant coronary artery in 50% of the general population is the right coronary. In addition, 10% to 15% of the general population are left coronary dominant, and 35% to 40% of the general population have mixed right and left dominance.
Venous Drainage
An extensive venous system exists in the heart. The three major systems include the coronary sinus, the anterior cardiac veins, and the thebesian veins (Figure 23-6).
The coronary sinus is located in the posterior AV groove near the crux. It collects approximately 85% of the blood from the LV, and for this reason it is catheterized when metabolic studies of the LV are performed. It may also be cannulated during cardiopulmonary bypass to deliver cardioplegia. The coronary sinus receives blood from the great, middle, and small cardiac veins; the posterior left ventricular veins; and the left atrial vein of Marshall.
Cardiac Innervation
Increased sympathetic nervous system tone increases heart rate (chronotropic), force of myocardial contraction (inotropic), and rate of sinus node discharge (dromotropic). Sympathetic nervous system activation results in the mobilization of myocardial fat-free acids and glycogen for energy use by the myocardial cells. The preganglionic sympathetic nervous system fibers originate from the cells in the intermediolateral columns of the higher thoracic segments of the spinal cord and synapse at the first through the fourth or fifth thoracic paravertebral ganglia. These spinal cord segments are known as the cardioaccelerator fibers. The postganglionic fibers then travel as the superior, middle, and inferior cardiac nerves and the thoracic visceral nerves. These fibers form an epicardial plexus and are distributed over the entire ventricular myocardium. There is greater distribution of sympathetic nerves that innervate the ventricles, resulting in increased ventricular contractility, as is shown in Figure 23-7. Catecholamines released during sympathetic stimulation bind with adrenergic receptors on the heart (primarily B1) and change the biochemical properties within the myocyte. This process is discussed later in this chapter.
The preganglionic parasympathetic fibers originate in the dorsal motor nucleus of the medulla. Short postganglionic fibers primarily innervate the SA and AV nodes and the atrial muscle fibers (see Figure 23-7). For this reason, increased parasympathetic tone decreases heart rate (HR). The function of the parasympathetic nervous system is primarily to slow the HR and secondarily to decrease contractility. In fact, maximal vagal (parasympathetic) stimulation reduces contractility by only 30%, whereas maximal sympathetic stimulation increases contractility by 100%. Acetylcholine is the neurotransmitter of the parasympathetic nervous system. Acetylcholine binds to muscarinic receptors on the heart and decreases the rate of sinus node discharge and slows conduction velocity through the AV node. The physiologic effects of parasympathetic nervous system stimulation occur because of increased permeability of cardiac muscle cell membranes to potassium, resulting in hyperpolarization. As a result, SA and AV node cells are less excitable.
Cardiac Conduction System
Within the myocardium lies the specialized conduction system whose purpose is to automatically initiate and coordinate the cardiac rhythm. The cells of this system differ from the other myocardial cells because they are more variable in shape, contain fewer myofibrils, and have a characteristic pale staining of the cytoplasm. The conductive system consists of the following components: the sinoatrial (SA) node, the internodal tracts, the AV node, the AV bundle, and the Purkinje system (Figure 23-8).
Sinoatrial Node
The SA node (the Keith-Flack node) is a small mass of specialized cells and collagenous tissue located along the epicardial surface at the junction of the superior vena cava and the RA. It has a prominent central artery that is a branch of the right coronary artery. The SA node is derived from the junction of the right horn of the sinus venosus and the primitive atrium. The SA node consists of two cell types: P cells (pacemaker cells), which are pale and ovoid with large round nuclei, and intermediate or transitional cells, which are elongated. These transitional cells are intermediate between ovoid and ordinary cells. They conduct impulses within and away from the SA node.
Structural and Regulatory Proteins
The myocardial cell is similar to skeletal muscle in that it is composed of sarcomeres (Figure 23-9). These sarcomeres contain all the microfilaments and structures that are consistent with the skeletal muscle sarcomere. The sarcomere stretches from Z line to Z line. The A bands consist of the actin filaments, which contain a bilayer filament of F-actin and tropomyosin. Along the actin filament, many active sites exist that can attach to the head of the myosin molecule. A troponin complex is necessary to inhibit actin and myosin from interacting and initiating muscle contraction. The other microfilament in the cardiac muscle is the myosin molecule. This molecule is made up of two major parts: a light meromyosin chain and a heavy meromyosin chain. The heavy meromyosin chain consists of two hinged ends and a head that plays a role in the “ratchet theory” of muscle contraction (Figure 23-10).1
Evidence indicates that the troponin-tropomyosin complex inhibits the binding of the heads of the myosin filaments with the active sites on the actin molecule (Figure 23-11). During the initiation of contraction, calcium is released from the sarcoplasmic reticulum. Calcium binds to the troponin-tropomyosin complex and causes a conformational change so that the active binding sites on the actin filaments become exposed. The myosin cross bridges bind to the active filament and move along the actin filament by alternately attaching and detaching from the active sites, thereby causing shortening of the Z lines (Figure 23-12). This is known as the sliding filament theory. When the actin filaments and myosin cross bridges intermingle, muscle contraction occurs. Inhibition of calcium influx into the cardiac muscle cells is the proposed mechanism whereby the inhaled anesthetic agents cause depression of myocardial contractility. Cellular energy or adenosine triphosphate (ATP) is required for this process, known as excitation-contraction coupling, to occur, as shown (Figure 23-13). For muscle contraction to cease, calcium reuptake into the sarcoplasmic reticulum occurs as a result of active transport. The troponin-tropomyosin complex reinhibits the interaction between actin and myosin. That this process occurs constantly and within milliseconds is the primary reason for the heart’s high metabolic demands (see Figure 23-13). With a limited supply of oxygen and substrate (primarily fatty acids), such as with patients with coronary artery disease or an increased energy demand caused by tachycardia from sympathetic nervous system stimulation, myocardial dysfunction and infarction can occur.
Clinically this concept is demonstrated by having the ideal filling pressure of the LV necessary to achieve adequate CO. Filling pressures are used to reflect the filling volumes of the ventricles and (indirectly) the amount of stretch on the ventricular muscle at rest. Filling pressures are measured by the use of the pulmonary capillary wedge pressure (PCWP) or the pulmonary artery diastolic pressure. It has been demonstrated that at excessively high filling pressures (as in congestive heart failure) and at excessively low filling pressures (as in hypovolemia), the CO can be compromised as a result of either excessive or inadequate stretch of the left ventricular myocardium. The greater the degree of stretch of myocardial muscle fibers, the greater the number of actin filaments and myosin cross bridges that are more completely approximated. This will result in an increase in the force of cardiac contraction, as shown in Figure 23-14. This concept is the basis for the Frank-Starling law of the heart, which is discussed later in this chapter.
Differences Between Skeletal and Cardiac Muscle Cells
Several differences exist between myocardial muscle cells and skeletal muscle cells (see Figure 23-9). At the junctions between the fibers in the myocardial muscle mass, many branching, interconnected fibers are intercalated disks and gap junctions, or nexi. Areas of low resistance facilitate the conduction of the action potential from one myocardial cell to another.
Generation of Membrane Potentials
Resting Membrane Potentials
The chemical force relies on the potential difference in ion concentration between one side of the cell membrane and the other. The ions primarily responsible for this force are sodium, potassium, and calcium.13 The electrostatic counterforce results from the negative potential generated by the ion difference of the interior of the cell. This force can pull ions into the cell, especially potassium.
Calculation of the equilibrium potential (Em, measured in millivolts) has been accomplished by examining the concentration of an ion inside the cell versus outside the cell (see the Nernst equation that follows). Table 23-1 lists the equilibrium potentials of the most physiologically important ions. The ion most responsible for the resting membrane potential is potassium.
where R is a gas constant, T is temperature in Kelvin, F is Faraday’s constant, [K]i is the intracellular concentration of potassium, and [K]o is the extracellular concentration of potassium.
where [K]i is the intracellular ion concentration of potassium, [K]o is the extracellular ion concentration of potassium, PNa is the membrane permeability of sodium, PCl is the membrane permeability of chlorine, and PK (when calculated) is the membrane permeability of potassium.
Ventricular Muscle Fiber Action Potential
Phases of the Action Potential
The action potentials of the various parts of the conduction system vary according to their locations and functions.14 The action potential of the ventricular muscle fiber is separated into five phases (Figure 23-15). Phase 0, or upstroke, is represented by depolarization and involves the fast sodium channels. The fast sodium channel activation gates (M gates) open between −70 and −65 mV (threshold potential). At 0 mV, both the activation and the inactivation gates (H gates) are open. The rapid upstroke velocity of phase 0 gives a relative indication of the conductivity of the myocardial cell. Local anesthetics such as lidocaine have an inhibitory effect on phase 0 by decreasing the influx of sodium. Table 23-2 describes the phases and events of a cardiac action potential.
One of the characteristics of the action potential unique to ventricular muscle is phase 2, or the plateau phase. The plateau phase exists because the slow calcium channels open at −30 to −40 mV and allow an influx of calcium. This inward calcium flux delays repolarization and prolongs the absolute refractory period. Toward the end of phase 2, a decreased permeability to potassium occurs that accounts for a small outward leakage of potassium balanced by the calcium and sodium influx that maintains a membrane potential near 0 mV. Calcium channel blockers exert their pharmacologic effect during phase 2. The physiologic effects include decreased contractility, decreased heart rate, and decreased cardiac conduction velocity.15
The sodium-potassium pump, which is dependent of ATP, reestablishes the proper intracellular-to-extracellular ionic concentrations during phase 4 (diastolic repolarization phase). Phase 4 lasts from the completion of repolarization to the next action potential. Lidocaine lengthens the duration of phase 4 by decreasing the cardiac cell membrane’s permeability to potassium ion, thereby decreasing the efflux of potassium and delaying the onset of the resting membrane potential.15
The cardiac glycoside digoxin inhibits the sodium-potassium ATP-dependent pump, decreasing sodium efflux into the extracellular fluid. As intracellular sodium concentrations increase, the exchange between sodium and calcium is decreased. The result is a higher concentration of calcium remains within the cardiac cell, and this effect is believed to be responsible for the increased inotropic effect.15
Sinoatrial Node Action Potential
The myocardium has among its characteristics contractility, automaticity, and conductivity. Each of the various myocardial masses has its own intrinsic automaticity and rate of action-potential initiation. The SA node is the primary pacemaker of the heart and has several unique characteristics (Figure 23-16). As a result of its higher resting membrane potentials, the SA node membrane is more permeable to sodium than other atrial myocardial cells. This “leakiness” gradually raises the membrane potential closer to threshold potential (−55 to −60 mV), at which point an action potential may be initiated. Therefore the action potential originating within the SA node differs from the action potential generated within the ventricular muscle mass. For this reason, the SA node is the primary pacemaker of the heart.
Physiology of the Heart
Cardiac Cycle
To understand the cardiac cycle, one must have a firm understanding of the basics of the anatomy of the heart and the pressures and volumes generated within the various chambers during the cardiac cycle. An appreciation for the valves and their positions during the phases of the cycle is essential (Figure 23-17). Additionally, notice that the electrocardiogram impulse generation precedes the mechanical action of the heart. This delay between the electrical impulse and the mechanical event occurs because time is needed for the wave of depolarization to spread across the myocardium before contraction can begin. In relation to the electrocardiogram, the P wave represents atrial systole, the QRS complex signifies ventricular systole, and the T wave represents ventricular repolarization.
Diastole: During ventricular systole, the atria fill and blood returns from the venous system to the right side of the heart and from the pulmonary circulation to the left side of the heart. The first phase of diastole is the period of isovolumetric relaxation. The ventricular muscle mass relaxes, and the aortic and mitral valves are closed as long as the ventricular pressure remains higher than the atrial pressure. The true filling phase is divided into three periods: (1) rapid inflow, or diastasis, (2) reduced inflow, and (3) atrial systole. Once the ventricular pressure drops below atrial pressure, the mitral valve opens, and the period of rapid inflow to passively fill the ventricle begins. The second period of diastole is diastasis, in which minimal changes occur in volume and in pressure.
Systole: After atrial systole, the isovolumetric phase, or isovolumetric contraction, which is the phase at the beginning of ventricular contraction, occurs. The myocardial fibers shorten, and pressure is generated within the ventricle but only enough to close the mitral valve. Therefore during this period, an increase in left ventricular pressure occurs without a change in ventricular diastolic volume. Isovolumetric contraction begins with closure of the mitral valve and lasts until opening of the mitral valve.
Physiology of Coronary Circulation
Coronary Blood Flow: The rate of blood flow is determined by a change in the pressure within the vessel divided by resistance of the system. Alterations of the radius of a vessel change the flow to the fourth power of the radius. This phenomenon is an extension of Poiseuille’s law, which determines the flow of a fluid through a tube.
At rest, approximately 4% to 5% of the CO, or 225 mL/min of blood, passes through the coronary vasculature. Phasic changes have been documented during coronary blood flow. A greater amount of coronary flow in the LV occurs during diastole. During systole, left coronary artery blood flow ceases to the subendocardium due to compression of the subendocardial vessels by the myocardium; flow through the epicardial vessels is not affected during systole to this extent. The flow to the left coronary artery is greatest during diastole as a result of the decreased resistance to flow from decreased myofibril tension that occurs as the intracavitary pressure decreases (Figure 23-18).
Control of Coronary Circulation and Oxygen Supply and Demand: Coronary blood flow is regulated by intrinsic and extrinsic factors that affect coronary artery tone. Intrinsic factors include the anatomic arrangement and perfusion pressure of the coronary vessels. Extrinsic factors include compressive factors within the myocardium, as well as metabolic, neural, and humoral factors. Blood flow through the coronary circulation is primarily controlled by the factors that determine oxygen demand and oxygen supply. Myocardial oxygen supply is determined by arterial blood content, diastolic blood pressure, diastolic time as determined by HR, oxygen extraction, and coronary blood flow. Myocardial oxygen demand is determined by preload, afterload, contractility, and HR (Figure 23-19).
The factors that increase myocardial oxygen consumption (mo2) are listed in Table 23-3. Notice in Figure 23-19 that heart rate appears on both the supply (diastolic time) side and demand side of the balance. Increasing heart rate not only increases demand but also decreases diastolic time, which is when 80% to 90% of coronary filling and myocardial perfusion occurs. Increased heart rate is the most important factor that negatively affects m
o2. Doubling the heart rate doubles m
o2.16 This phenomenon most dramatically affects patients with coronary artery disease, because the supply of blood is compromised and may not be able to meet the oxygen demands caused by tachycardia. As a result, myocardial dysfunction or infarction can occur. By slowing HR and decreasing contractility, β-blocking medications increase supply and decrease demand, protecting the heart from ischemia.17
The normal physiologic parameters of the heart are given in Table 23-4. The determinants of mo2 include myocardial contractility, myocardial wall tension (preload), HR, and mean arterial pressure (MAP; afterload). Oxygen extraction is determined by measurement of the difference between the oxygen tension in the pulmonary arterial blood and that in the coronary sinus.
TABLE 23-4
Heart size | 230-280 g (female) |
280-340 g (male) | |
Coronary blood flow | 225-250 mL/min or 4%-7% total cardiac output |
Myocardial O2 consumption | 65%-70% extraction |
8-10 mL O2/100 g/min | |
Normal autoregulation | 60-140 mmHg (MAP) |
Coronary filling | 80%-90% during diastole |
Autoregulation: Under normal physiologic conditions, the coronary circulation, like other tissue beds in the body, exhibits autoregulation, which is the ability to maintain coronary blood flow through a range of MAPs by dilating or constricting. Coronary blood flow is maintained at a constant rate through a MAP range of 60 to 140 mmHg. When arterial blood pressure is less than or exceeds these pressure limits, coronary blood flow becomes pressure dependent. Therefore during hypotension, when the coronary arteries are maximally dilated, coronary blood flow is determined by the MAP minus the right atrial pressure.
The concept of “coronary steal” has emerged, especially in reference to the use of agents such as adenosine, nitroglycerin, and isoflurane. If vasodilator treatment is used in a patient who has both an ischemic area of the heart that is supplying a stenotic vessel with collateral flow and another area that has an intact autoregulated vessel, only the autoregulated vessel dilates further and has the ability to increase its flow. Constant maximal coronary artery dilation exists in an area where stenosis is present. Therefore only the areas of the heart with intact autoregulation respond to vasodilators and receive preferential flow over the stenotic area. The existence of this phenomenon is questionable. As long as adequate CPP is maintained, coronary steal and myocardial ischemia caused by isoflurane do not occur.18,19 A second factor that could result in this phenomenon is coronary steal–prone anatomy. This has been defined as complete occlusion of one coronary artery and at least 50% occlusion of a second coronary artery that supplies collateral blood flow to the area in which the complete occlusion exists.18 In addition, recent evidence suggests that the inhaled anesthetics isoflurane, desflurane, and sevoflurane produce myocardial protection during periods of ischemia in humans by decreasing the formation of free radicals, preserving myocardial ATP stores, and inhibiting increased intracellular calcium.19,20 This is referred to as anesthetic preconditioning.
Cardiac Output: Cardiac output is the amount of blood ejected from the LV during 1 minute. Comparing various CO values among several patients requires a method for calculating output in relation to the size of the patient. The CO is measured in liters per minute. Cardiac output is indexed, because a CO of 3.5 L/min may be adequate for a patient who is 5 feet tall and weighs 95 lb, but it is less than optimal for a patient who is 6 feet 7 inches tall and weighs 300 lb. The average CO is 5 L/min, and the average cardiac index (CI) is 2.5 L/min or more per square meter of body surface area (BSA). The formula for this relationship is CI = CO/BSA.
Right-sided heart pressures (central venous pressure) that are obtained clinically can be estimates of left ventricular volumes in patients with good left ventricular function.21
Contractility of the myocardium is the state of inotropy that is independent of either preload or afterload. It may be altered by many cardiovascular disease states. Factors such as rate of pressure changes over time (dP/dt [first derivative of pressure measured over time]), force-velocity or Starling ventricular function curves, pressure-volume loops, ejection fraction (EF), and velocity of circumferential fiber shortening have all been used to estimate contractility.
Ventricular function curves22 (Figure 23-20), define the relationship between the left ventricular filling pressure (left ventricular diastolic pressure, left atrial pressure, PCWP) and the left ventricular stroke work index (LVSWI), which is calculated by use of the following equation:
where SVI = CI/HR.
Each left ventricular function curve has a steep upstroke that has a plateau at higher filling pressures. To apply the Frank-Starling mechanism to Figure 23-20, notice in the “normal” and “hyperdynamic” curves that as pressure (horizontal axis) increases, so does LV output (vertical axis). However, at the top of these curves, there is a plateau where increasing the filling pressures no longer increases performance and can then decrease ventricular output. Symptoms may be elicited by either high or low filling pressures. On the “failure” curve, with compromised cardiac function, increases in filling pressures do not dramatically increase myocardial performance and can lead to cardiogenic shock. The clinical determination of LVSWI is worthwhile because it contains many of the factors that contribute to CO, and it gives measures of both systolic and diastolic performance.
Left ventricular pressure-volume loops have been mentioned before as conceptual models depicting phases of the cardiac cycle. They may also be used as tools to determine myocardial performance. Left ventricular pressure-volume loops simultaneously measure chamber pressures and the resultant volumes (Figure 23-21). Movement from left to right on the horizontal axis represents increased volume. Movement from right to left on the horizontal axis represents decreased volume. Movement up and down on the vertical axis represents increases and decreases in pressure, respectively. The distinct phases of the left ventricular pressure-volume loop are represented in Figure 23-22.
or
The EF is the percentage of the end-diastolic volume ejected during systole, as seen in Figure 23-23. The normal EF is 60% to 70%. An EF of less than 40% is associated with significant left ventricular impairment.
Deviations from the normal left ventricular pressure-volume loops occur as a result of many causes. Factors that alter the normal loop include increases and decreases in LV preload, LV afterload, and LV contractility. These factors can be acute and transient, such as during the administration of vasoactive medications, or chronic as a result of myocardial compensation caused by valvular heart disease. In Figure 23-24, these changes are consistent with valvular heart disease. Because many factors contribute to variations in pressure and volume from beat to beat within the LV, each pressure volume loop is distinct, represents one LV systolic ejection, and is different for each contraction. A discussion of these pathologic curves is presented later in this chapter.
Cardiovascular Reflexes
Valsalva Maneuver
The Valsalva maneuver occurs as a result of forced expiration against a closed glottis. The reflex is mediated through the baroreceptors located near the bifurcation of the internal and external carotid arteries (carotid sinus) and the aortic arch. The afferent pathway is directed via Hering’s nerve and either the glossopharyngeal nerve (carotid sinus) or the vagus nerve (aortic arch), as shown in Figure 23-25. Stimulation of either of these areas inhibits the vasomotor center in the medulla. The response inhibits the sympathetic nervous system and stimulates the parasympathetic nervous system, producing a decrease in HR, a decrease in myocardial contractility, and vasodilation, resulting in a decrease in blood pressure. The Valsalva maneuver also increases intrathoracic pressure, which decreases venous return and thereby decreases CO.
Baroreceptor Reflex
The baroreceptors respond to fluctuations in arterial blood pressure. Afferent and efferent impulse transmission travels along the same pathway as the Valsalva maneuver. Decreases in arterial blood pressure are sensed by the baroreceptors, increasing sympathetic tone, which results in increased myocardial performance and vasoconstriction. Acute hypertension causes the opposite cardiovascular response. A more in-depth explanation of the baroreceptor response and its role in short- and long-term blood pressure control is presented later in this chapter. The baroreceptor response is inhibited by volatile anesthetic agents in a dose-dependent manner and results in decreased ability of the baroreceptors to respond to blood pressure changes when these agents are used.
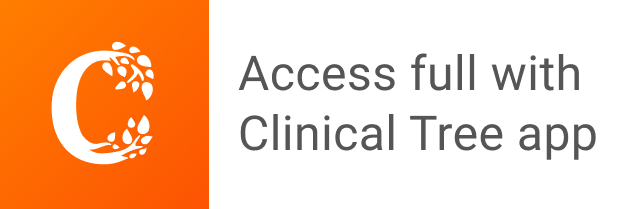