© Springer International Publishing AG 2018
Ehab Farag, Maged Argalious, John E. Tetzlaff and Deepak Sharma (eds.)Basic Sciences in Anesthesiahttps://doi.org/10.1007/978-3-319-62067-1_1111. Cardiovascular Anatomy and Pharmacology
(1)
Department of Anesthesiology and Critical Care Medicine, The Johns Hopkins Hospital, Baltimore, MD, USA
11.2.2 Antiarrhythmic Agents
11.2.4 Inotropes and Vasopressors
11.2.5 Antihypertensive Agents
11.2.8 Digitalis
11.2.9 Questions and Answers
Keywords
Cardiac anatomyCardiovascular pharmacologyElectrophysiologyAntiarrhythmic agentsPulmonary hypertensionIvabradineNesiritideARNIKey Points
- 1.
The major determinants of myocardial O2 supply are coronary blood flow and arterial O2 content. Coronary blood flow is determined by the patency of the coronaries, coronary perfusion pressure, and coronary vascular resistance.
- 2.
The major determinants of myocardial O2 demand are heart rate, inotropic state, and wall tension (which is the function of intracavitary pressure, radius and wall thickness, preload and afterload).
- 3.
Critical O2 delivery is the point at which the extraction ratio is maximized, and any further incongruence between demand and supply will lead to tissue hypoxia.
- 4.
In the perioperative setting, continuation of long-standing beta blocker therapy is recommended.
- 5.
Beta blockers should not be started on the day of surgery in beta blocker naïve patients.
- 6.
Patients with coronary artery disease undergoing surgical coronary revascularization should be administered a beta blocker before surgery.
- 7.
Although recent perioperative guidelines suggested continuing angiotensin converting enzyme (ACE) inhibitors/angiotensin receptor blockers (ARBs) before non-cardiac surgery, adverse circulatory effects during anesthesia in patients chronically treated with ACE inhibitors/ARBs has led to the recommendation that these drugs be discontinued 24 h before surgery.
- 8.
Epinephrine inhibits uterine contractions especially during the second stage of labor.
11.1 Part 1: Cardiac Anatomy
11.1.1 Development and Anatomy of the Heart
The heart is a conical hollow muscular organ located between the lungs in the mid-mediastinal portion of the thorax, suspended in the pericardial sac. As a dual pump, it maintains unidirectional blood flow to the body and the lungs by its rhythmical torsion and untwisting throughout the series of cardiac cycles. Its receiving chambers (the left and right atrium, composed of 2 myocardial layers), and its pumping chambers (the left and right ventricle, composed of 3 layers of myocardium building up the 2 separate vortices each ventricle) are structurally separated by their respective interchamber septa corresponding with the long axis of the heart, and are electrically isolated by the left and right fibrous rings comprising the fibrous cardiac skeleton in the short axis of the heart, perpendicular to the long axis.
A Brief Review of Cardiogenesis
The development of the cardiovascular system begins on day 17 of gestation, when mesoderm-derived blood islands, consisting of endothelial cells and hemoblasts, begin to form. These blood islands coalesce to form a pair of endothelial heart tubes, which on day 21 fuse into a single primitive heart tube with a cranial inflow (arterial) and a caudal outflow (venous) end. This primitive heart tube is divided into 5 regions: truncus arteriosus, bulbus cordis, primitive ventricle, primitive atrium, and sinus venosus. Initial contractions occur on day 21–22, and unidirectional circulation is established by week 4. From weeks 4–7, folding and septation of the heart and the great vessels takes place, critical to the development of the 4 chambers and the normal embryonic vascular circuit. In the developed heart, the atrial chambers lie cephalad and to the right of their corresponding ventricles, and the right-sided chambers lie anterior to their corresponding left-sided chambers. The atrial and ventricular septation is summarized later.
A Review of the Gross Anatomy of the Heart
Fibrous Skeleton
The fibrous skeleton of the heart is a framework of 4 dense collagen rings around the atrioventricular (AV) and semilunar valves, as well as the left and right fibrous trigones (also known as the central fibrous body), and the membranous portion of the interatrial and interventricular septum. Its main component is the central fibrous body, where the leaflets of all 4 cardiac valves converge. The fibrous skeleton reinforces the ostia of the valves and prevents the annuli from overdistension by resisting forces of pressure developing through the cardiac cycle. It provides attachment for the valvular leaflets and cusps, as well as for some of the musculature of the atrial and ventricular wall. It is electrically impermeable, only allowing electrical propagation from the atrioventricular node across the right fibrous trigone to the bundle of His. Chronic degenerative changes and calcium deposition into the collagen skeleton results in delayed conduction and depolarization, arrhythmias, rigidity of the valvular ostia, restricted leaflet opening and/or leaflet malcoaptation.
Interatrial Septum
The interatrial septum is a blade-shaped wall between the left and right atrium. Its development begins during the fifth gestational week with the septum primum growing from the posterior wall of the primary atrium toward the endocardial cushion, formed within the atrioventricular canal. With the incomplete fusion of the septum primum and the endocardial cushion, a progressively diminishing space above the endocardial cushion, the ostium (also known as foramen) primum remains. During this process, small coalescing perforations in the upper portion of the septum primum create the ostium (or foramen) secundum. Concurrently, a second septum, the septum secundum begins to form to the right of the septum primum, growing from the anterior wall, partially covering the foramen primum. Its growth stops at the seventh week of gestation, leaving a gap known as the foramen ovale. This is essential in reducing pulmonary blood flow through the inactive fetal lungs.
The foramen ovale is continuous with the ostium secundum, and is covered by the septum secundum on its left side. As a result of normal adaptive changes in the neonatal circulation, the decreasing pulmonary vascular resistance (PVR) and the resultant reversal of interatrial pressure gradient results in the permanent functional closure of the foramen ovale. In one-third of the population incomplete fusion between the septum primum and the septum secundum results in a residual flap valve and a probe patent foramen ovale (PFO).
Excessive apoptosis within the septum primum, or incomplete development of the septum secundum results in an atrial septal defect (ASD), the most common congenital heart defect to manifest in adulthood:
-
There are 4 types of ASD, the most common being the secundum type ASD, accounting for 80–90% of all ASDs. The secundum type ASD is located centrally, it is larger than a PFO, and it is commonly associated with mitral valve prolapse.
-
The primum type ASD is less common, it represents 2–3% of ASDs. It is located in the lower portion of the interatrial septum, and it is associated with endocardial cushion defects, AV-valve abnormalities, or ventricular septal defects such as in Down syndrome.
-
The sinus venosus ASD represents 2–10% of ASDs. It is associated with abnormal pulmonary venous return.
-
The fourth type, the coronary sinus ASD or unroofed coronary sinus is the least common. It results from incomplete septation between the inferior portion of the left atrium and the coronary sinus, and is commonly associated with a persistent left superior vena cava.
ASDs often remain clinically silent with preserved normal left atrial size, however, longstanding left-to-right shunting and resultant dilatation of the right-sided chambers along with persistent pulmonary hypertension may reverse the direction of the shunt, resulting in hypoxemia and Eisenmenger physiology.
Interventricular Septum
The interventricular septum (IVS) separates the left and right ventricles from one another. Under physiological pressure and filling conditions its convexity is bowing into the right ventricle. Its margins are marked externally by the anterior and posterior interventricular grooves. The upper, posterior part of the interventricular septum is thin, and constitutes the membranous interventricular septum. The greater, anterior portion is the muscular interventricular septum.
During cardiogenesis, along with the atrial septation a concurrent ventricular septation is also taking place. With the development of the endocardial cushions and the atrioventricular separation, there is tissue growth between the left and the right sides of the developing atrioventricular canal. This is the muscular portion of the interventricular septum, growing from the inferior portion of the ventricle toward the endocardial cushion. As the muscular IVS reaches the endocardial cushion, a small interventricular foramen remains. This is closed by tissue growth from the endocardial cushion, connective tissue from the muscular IVS, as well as tissue from the septation of the truncus pulmonalis and the conus arteriosus. Inadequate contribution from either component results in different types of ventricular septal defects.
Chambers and Valves of the Right Heart
Right Atrium
The right atrium is the cardiac chamber receiving the systemic and cardiac venous return via the superior and inferior venae cavae (SVC and IVC) and the coronary sinus. It is divided into 3 components.
The venous component receives deoxygenated blood from the SVC and the IVC. At the inferior cavoatrial junction lies the Eustachian valve, important before birth in directing blood from the IVC to the left atrium across the foramen ovale. A network of fine filamentous strands, the Chiari network, may be seen in this region. The variably sized Eustachian valve and Chiari network are normal variants within the right atrium.
The second component is the vestibule, which converges into the leaflets of the tricuspid valve, and the third is the appendage (also known as auricle). The right atrial appendage has a wide junction with both the vestibule and the venous component. The vestibuloauricular junction is identified by the terminal groove, the external marking of the prominent terminal crest on the internal surface. A consistent feature of the right atrial anatomy is the extension of the auricular pectinate muscles beyond the appendage to the atrioventricular junction.
The sinus node, a cluster of specialized myocardial cells capable of spontaneous electrical impulse generation, lies at the superior cavoatrial junction at the terminal groove. It is supplied by the nodal artery, arising from the right coronary artery in 55% of cases, or the proximal left circumflex artery in the remaining 45%. The atrioventricular node is located in the floor of the right atrium, near the opening of the coronary sinus. Preserving blood flow to the sinus node and other conductive tissues is key to avoiding arrhythmias and other conduction abnormalities.
Tricuspid Valve
The tricuspid valve is located nearly vertically behind the aortic valve. With a valve area of 4–6 cm2, it is the largest of the 4 valves in the heart. Its annular plane is saddle-shaped and is apically displaced relative to that of the mitral valve. A displacement index greater than 8 mm/m2 (body surface area) suggests the presence of Ebstein’s anomaly. Its 3 leaflets are the septal, anterior, and posterior tricuspid valve leaflets, attached by their chordae tendineae to the right ventricular papillary muscles (true chords), or, not uncommonly, directly to the septum (false chords). The subvalvular apparatus of the tricuspid valve is variable. Most commonly, the chordae tendineae originate from 2 or 3 papillary muscles, the anterior being the most prominent; the posterior, which is usually less prominent and may have several subdivisions; and the septal, which is the least prominent or may be entirely absent.
Right Ventricle
The right ventricle is the most anterior of the 4 chambers of the heart, located immediately behind the sternum. Its geometry is complex: The right ventricle is crescent shaped when viewed in the short axis, and triangular when viewed longitudinally. Current guidelines identify its walls as anterior, inferior, and lateral free wall. Medially, the right ventricle shares the septum with its left-sided counterpart. For purposes of functional quantification, these walls are further divided into basal, mid, and apical segments.
Anatomically and functionally, the right ventricle is composed of 3 portions: the smooth muscular right ventricular inflow tract, the apical trabecular, and the right ventricular outflow tract. The inlet portion encircles the tricuspid valve. The trabecular portion of the right ventricle extends into the apical region. The wall of the ventricle is thin here, making it vulnerable to perforation by catheters and electrodes.
The most prominent of the trabeculae is the septomarginal trabecula. It carries part of the right bundle branch of the conduction system. It was once wrongly thought to prevent the right ventricle from overdistending, hence the name “moderator band.” The septomarginal trabecula originates from the base of the septum, it divides into an anterior limb that supports the pulmonic valve cusps, and a posterior limb, from which the medial papillary muscle arises. Its main mass extends from the base of the septum to the apex and divides into smaller muscle ridges. Two of these are particularly prominent, one giving rise to the anterior papillary muscle, and the other crossing the right ventricular cavity as the moderator band.
The outlet portion consists of the circumferential muscular infundibulum that supports the pulmonic valve, which is the only one of the cardiac valves with no single ringlike annulus. It is supplied by the conus branch of the right coronary artery.
Pulmonic Valve
The pulmonic valve separates the right ventricular outflow tract from the pulmonary artery. It is located anteriorly and to the left of the aortic valve. It is a semilunar valve formed by 3 cusps, labeled as anterior (nonseptal), left, and right, each with a fibrous nodule at the midpoint of their free edges. The leaflets coapt via their crescent-shaped surfaces. The cusps of the pulmonic valve are formed by endocardial folds that are supported by internal dense collagenous plates as well as elastic connective tissue, continuous with the fibrous skeleton of the heart. They are thinner than the cusps of the aortic valve, and, lacking fibrous continuity with the septal leaflet of the tricuspid valve, are supported only by a prominent ridge of the posterior subpulmonary infundibular musculature. This is the supraventricular crest that separates the pulmonic and tricuspid valves from one another, and supports the semilunar attachments of the pulmonic valve. Surgical incisions made through this crest may run toward the interventricular septum, and jeopardize the right coronary artery.
Chambers and Valves of the Left Heart
Left Atrium
Oxygen-rich blood returning from the lungs via the 4 pulmonary veins is received first by the left atrium. The left atrium has 3 basic components: first, the largest, smooth-walled pulmonary venous component; second, the vestibule; and third, the left atrial appendage. The wall of the vestibule is continuous with both the venous component and the posterior (mural) leaflet of the mitral valve, and may affect normal valvular function when left atrial dilation is present. Pectinate muscles are confined almost exclusively to the appendage in the left atrium. The flap valve of the fossa ovalis is found on the left atrial side of the interatrial septum.
Recurrent, symptomatic, drug-refractory atrial fibrillation most often originates from the pulmonary veins or their pulmonary venous ostia. Circumferential or segmental ablation of potential triggers is considered effective means to control refractory atrial fibrillation, as scar forming is thought to prevent propagation of abnormal signals to the rest of the atrial musculature. The ablation and mapping catheters are inserted via the femoral or jugular veins, and are placed through separate transseptal punctures. Most often, radiofrequency energy is used to make ablation lesions around the pulmonary vein ostia. In the presence of a left atrial appendage thrombus, or inability to anticoagulate in the 30-day peri- and post-procedural period, pulmonary vein isolation for atrial fibrillation ablation is contraindicated. A rare, frequently disabling, sometimes fatal complication of the procedure is an atrio-esophageal fistula resulting from thermal damage. Other potential complications are cardiac tamponade, arrhythmias or atrioventricular block, embolic events, valvular complications, or peripheral vascular damage.
Mitral Valve
The bileaflet mitral valve is located between the left atrium and left ventricle, embedded into the saddle-shaped mitral valve annulus. It serves as a unidirectional valve directing blood from the atrium toward the ventricle by passively opening during diastole and closing in systole, as determined by the pressure gradient between the chambers.
The mitral valve apparatus consists of the left atrial wall, the annulus, the anterior leaflet attached to the aortic root, the posterior leaflet attached to the left atrial myocardium, the chordae tendineae attached to the ventricular side of the mitral valve leaflets, and the anteromedial and posterolateral papillary muscles.
The anterior leaflet of the mitral valve is wide. It occupies about one-third of the annular circumference, and forms the anterior portion of the left ventricular outflow tract. In contrast with the posterior leaflet, its free edge has no indentations. The posterior (mural) leaflet is narrower, it occupies about two-thirds of the annular circumference, and it is further divided into 3 scallops by 2 clefts. Because the posterior aspect of the mitral valve annulus contains little fibrous tissue, the posterior leaflet, especially the middle scallop, is more prone to prolapse than the anterior. The circumflex artery courses near the left half of the posterior leaflet, whereas the right half is in close proximity to the coronary sinus and the arterial branch supplying the AV node.
The mitral valve leaflets are supported by a dense collagen annulus. The most vulnerable portion of the mitral valve annulus is its aspect continuous with the right fibrous trigone, due to the proximity of the atrioventricular node and the penetrating bundle of His.
The muscular components of the mitral valve apparatus are the anteromedial and posterolateral papillary muscles. The anteromedial papillary muscle is more prominent and is supplied by the left coronary system. The posteromedial papillary muscle is smaller, and is supplied by the right coronary artery. The mitral valve closes with the contraction of the papillary muscles, and open when they relax. Papillary rupture, as a result of ischemia, will result in acute mitral regurgitation. Because the anterolateral papillary muscle is supplied by both the left anterior descending (LAD) artery and the left circumflex artery, its ischemic dysfunction is relatively uncommon. In about 60–65% of individuals, the posteromedial papillary muscle was found to be perfused by a single vessel, most commonly by the right coronary artery.
Left Ventricle
The left ventricle (LV) is the largest and thickest chamber of the heart. It receives oxygenated blood from the left atrium during diastole, to transfer it to the body during across the aortic valve during systole. Relative to the right ventricle, it is located laterally and posteriorly. Its superior, widest aspect is termed the base, leaning upward and toward the right shoulder, opposing its apex, pointing down and to the left. By consensus, its 4 walls are termed septal, and the free anterior, inferior, and lateral walls. For purposes of functional quantification, these are further divided into basal, mid, and apical segments.
Morphologically, the left ventricle also has an inlet, a fine trabecular and an outlet component. The inlet portion extends from the mitral valve annulus to the origins of the papillary muscles, and contains the mitral valve apparatus. In contrast to the coarsely trabeculated right ventricle, the left ventricular free wall is finely trabeculated. The trabeculation extends to the apex, where, due to the progressively decreasing number of myocardial fibers, the wall thickness is thin. Its outflow tract differs from that of the right ventricle in that it is anatomically and functionally contiguous with the inlet via the aortic-mitral continuity. The left ventricular outflow tract supports the aortic valve, and its septal portion, in contrast with the primarily muscular right ventricular infundibulum, includes the membranous interventricular septum. The left bundle branch of the conduction system courses posteriorly to the membranous septum between the right and noncoronary cusps of the aortic valve.
The interventricular septum is formed by subendocardial myocardial fibers of both the left and the right ventricle, intermingled with circumferentially arranged fibers derived from the left ventricle. For this reason, in a normally functioning heart, the septum thickens toward the left ventricle during systole. During contraction, the high left ventricular pressure along with the septum provides a “splint” against which the right ventricle is able to shorten, hereby contributing to the emptying of the right ventricle (systolic ventricular interdependence). Under pathologic conditions, for example, during acute right ventricular volume or pressure overload, or due to conduction abnormalities or pacemaker activation, the septum may appear flat, or move paradoxically toward the right ventricle during systole. Blood flow to the anterior 2/3 of the septum is supplied by the septal perforators of the left anterior descending artery. The posterior 1/3 is supplied by the distal branches of the right coronary artery.
The 2 papillary muscles of the left ventricle arise from the anterolateral and posteromedial portions of the left ventricular free wall. Their arrangement ensures parallel alignment of the chordae tendineae with the LV axis during systole, allowing for complete leaflet coaptation and closure of the mitral valve in the normally functioning heart.
All 3 coronary branches contribute to the blood supply of the left ventricle, acute ischemic events therefore predictably manifest as regional wall motion abnormalities based on the coronary distribution. The left anterior descending artery and its branches, the septal perforators and the diagonals perfuse the anterior wall, the anterior two-thirds of the septum, and the apex. The obtuse marginal branches of the left circumflex artery supply the lateral wall. The right coronary artery perfuses the medial portions of the inferior wall, as well as the posterior one-third of the interventricular septum. Anastomotic connections between the left and the right coronary system allows for preserved myocardial perfusion distal to a significant obstruction or total coronary occlusion.
Aortic Valve
The aortic valve is part of the aortic root consisting of the annulus, the aortic valve cusps, the sinuses of Valsalva, the junction between the sinuses and the proximal ascending aorta (the sinotubular junction), and the proximal ascending aorta. It is located at the left ventricular outlet, separating the aorta and the ventricle. Owing to its central location, it is the only valve in the heart that is related to all 4 chambers and each of the valves. As a trileaflet semilunar valve, it consists of 3 cusps: the left and right coronary cusps giving rise to their respective coronary arteries, as well as the non-coronary cusp, which faces the interatrial septum. The right coronary cusp is the most anterior and is adjacent to the right ventricular outflow tract. The left coronary cusp is located posteriorly relative to the other 2. Histologically, its cusps are composed of dense collagenous plates, which are in continuity with the fibrous skeleton of the heart, covered by endocardium. Each cusp has its attachment to the aorta and the left ventricle. Immediately behind the cusps, the wall of the proximal ascending aorta bulges outward to form the aortic sinuses of Valsalva. The function of these sinuses is to prevent coronary ostial occlusion by creating eddy currents of blood flow during rapid ejection. As with the pulmonic valve, each cusp has a fibrous nodule at the midpoint of their free edges, forming the nodule of Arantius. The leaflets coapt via their crescent-shaped surfaces. Incomplete coaptation of the valve leaflets during diastole results in aortic regurgitation, whereas limited systolic leaflet excursion due to excessive leaflet thickening resulting from degenerative or rheumatic disease process suggests the presence of aortic stenosis. The severity of aortic stenosis can be assessed by various methods. A peak transaortic velocity of greater than 4 m/second, a peak transaortic gradient of at least 70 mm Hg and a mean gradient of at least 40–50 mm Hg, as well as an aortic valve area of less than 1 cm2 is consistent with severe aortic stenosis.
Coronary Circulation
The first pair of arteries branching off the proximal ascending aorta are the left and right coronaries, originating from their respective sinus of Valsalva in the aortic root. While there is some variability in their point of origin and their distribution, the right coronary artery (RCA) almost always supplies the right ventricle (RV), whereas the left coronary artery (LCA) supplies the anterior and lateral wall of the left ventricle (LV), as well as the anterior portion of the interventricular septum. Blood supply to the remainder of the left ventricle is determined by the coronary dominance. This refers to the artery that supplies the posterior descending artery (PDA) and the posterior lateral branch (PLB). Right dominance (when the right coronary artery gives off the PDA and the PLB) occurs in 80–90% of normal individuals. Left dominance (the PDA and the PLB originating from the left circumflex artery) is slightly more common in men than in women. If the PDA is supplied by the RCA and the PLB branches off the left circumflex artery, the system is codominant.
The Left Coronary System
The left coronary artery arises from the left coronary sinus as the left main stem, which then bifurcates into 2 segments: the left anterior descending (LAD) and the circumflex (LCx) artery. The relatively short left main stem courses between the left atrial appendage and the pulmonary trunk before giving off the LAD and the LCx, and, in 15–30% of cases the variant coronary ramus intermedius, the branch supplying the anterior aspect of the heart.
The left anterior descending artery traverses the anterior interventricular groove and perfuses the anterior wall via the diagonal branches, as well as the anterior two-third of the interventricular septum via its septal perforators coursing along the anterolateral wall, and a small portion of the anterior wall of the right ventricle, adjacent to the interventricular groove, via the right ventricular branches.
The circumflex artery courses in the left atrioventricular groove. Its primary branches are the obtuse marginals, supplying the lateral aspect of the left ventricle, including the posteromedial papillary muscle. In 10% to 15% of individuals, it gives off the posterior descending artery (left dominance). Further branches of the left circumflex artery supply the left atrium, and, in 45% of the cases, the sinus node. A left dominant coronary system also supplies the atrioventricular node.
The Right Coronary System
The right coronary artery emerges from the right sinus of Valsalva and is carried by the right atrioventricular groove. In about half of the cases it gives off the conus branch that supplies the right ventricular outflow tract. The RCA perfuses the anterior free wall of the right ventricle via its acute marginal branches, the sinus node via the nodal artery in 55% of individuals, and the atrioventricular node in patients with right-dominant coronary circulation. It bifurcates into the posterior descending artery and the posterolateral branch at the posterior interventricular groove. The posterior descending artery gives off the posterior septal perforators that supply the posterior one-third of the interventricular septum. The posterolateral branch supplies the inferoposterior wall of the left ventricle along with the acute marginal branches of the RCA and/or the circumflex artery. The Kugel’s artery is an anastomotic branch between the RCA and the LCx that may give off a branch that supplies collateral circulation to the atrioventricular node.
The Coronary Veins
Of the cardiac veins, the great cardiac vein courses in the anterior interventricular groove upward from the apex, alongside the LAD. It drains into the coronary sinus. The middle cardiac vein courses in the inferior interventricular groove, upward from the apex, accompanying the PDA.
The coronary sinus courses in the posterior atrioventricular groove, alongside the left circumflex artery. It receives the great cardiac vein proximally and the middle cardiac vein distally, and drains into the right atrium. Its orifice is guarded by the Thebesian valve.
The anterior right ventricular veins course on the anterior surface of the right ventricle, where they may drain directly into the right atrium, or coalesce to form the small cardiac vein. The small coronary vein runs posteriorly through the right AV groove.
The Thebesian veins are small veins draining directly into the cardiac chambers, primarily into the right atrium and the right ventricle.
11.2 Part 2: Cardiovascular Pharmacology
11.2.1 An Overview of Subcellular Mechanisms: Ion Channels and Receptors
Patients in the perioperative period often receive agents that affect hemodynamic variables such as heart rhythm and rate, blood pressure, or cardiac output. The effect of these agents is governed predominantly by transmembrane ion fluxes. Drugs used for rhythm and rate control act by modulating Na+, K+, and Ca2+ currents. Intracellular calcium is a key mediator in coupling electrical excitation to mechanical contraction, and is an important determinant of the contractile state of the myocardium. The vascular tone of resistance vessels is regulated by ion fluxes via different types of K+, Ca2+ and Cl− channels.
Much of human physiology (for example, responses to stimuli by hormones, neurotransmitters, ions, or photons) is regulated by GTP binding (G) protein-linked signal transduction. G-proteins serve as points of communication between the extracellular and intracellular environment.
Adrenergic receptors are membrane-spanning molecules coupled to adenylate cyclase via a G-protein located on the inner membrane of the cell. Their activation ultimately leads to the modulation of downstream effectors. G-proteins consist of 3 (α, β and γ) subunits. The binding of an agonist to the adrenergic receptor replaces guanosine diphosphate (GDP) by guanosine triphosphate (GTP), and causes the α-subunit of the G-protein to break free from the β-γ complex, and act as a primary messenger: in beta receptors, it stimulates adenylate cyclase and triggers cyclic adenosine monophosphate (cAMP) production, which, as a second messenger in the process of signal transduction, activates its target kinases that phosphorylate regulator proteins and ultimately increases intracellular calcium levels.
Pure alpha-adrenergic agonists also increase intracellular calcium levels by stimulating phospholipase C, an enzyme that catalyzes hydrolysis of phosphatidyl inositol to diacyl glycerol and inositol triphosphate. Inositol triphosphate stimulates the release of calcium from the sarcoplasmic reticulum, and both molecules act as myofilamental calcium sensitizers.
11.2.2 Antiarrhythmic Agents
A Review of Electrophysiology and the Anatomy of the Cardiac Conduction System
Under physiologic conditions, electrical impulse generation, conduction, and propagation occurs in specialized excitatory and conductive tissues within the myocardium, and is driven by a sequence of ion fluxes through sarcolemmal ion channels. The pacemaker of the normal cardiac muscle is the sinoatrial (SA) node, located in the right atrium, below and lateral to the ostium of superior vena cava, supplied by the nodal branch of the right coronary artery (RCA). Its intrinsic rate (60–100 beats per minute) is determined by the resting transmembrane potential, the threshold potential, and the rate of phase 4 spontaneous diastolic depolarization. The SA node has the steepest slope of phase 4 depolarization. For this reason, its intrinsic rate is the highest, therefore this is the dominant, primary pacemaker of the heart.
Rhythmical, automatic impulses generated by the sinus node spread directly to the right atrium via its own non-contractile sinus fibers that fuse with excitable and contractile atrial fibers, as well as anteriorly to the left atrium via the Bachmann’s bundle. Electrical impulse from the right atrium propagates via 3 other internodal tracts, including the posterior (Thorel’s) and median (Wenkebach’s) pathways to the atrioventricular (AV) node. The AV node, supplied by the AV nodal branch of the distal RCA and the septal perforators of the left anterior descending (LAD) coronary artery, and located in the right atrial side of the interatrial septum behind the tricuspid valve and superior to the coronary sinus, delays impulses from the atria by approximately 70–100 ms before allowing them to pass to the bundle of His. These slow conduction velocities allow the atria to empty before ventricular contraction begins. The AV node is the primary regulator of ventricular rate in atrial fibrillation and flutter. Its intrinsic rate is 40–60 beats per minute, and it is considered a latent intrinsic pacemaker.
The bundle of His is located just above the interventricular septum (IVS) and is supplied by the septal perforators of the LAD, as well as the posterior descending artery. As a latent pacemaker, its intrinsic rate is 20–40 beats per minute. From the bundle of His, impulses rapidly travel down the bundle branches on either side of the membranous portion of the interventricular septum, to depolarize the left (left bundle branch, LBB, which also depolarizes the IVS) and the right (right bundle branch, RBB) ventricles. The left bundle branch further divides into the anterior and posterior fascicles. Both bundles terminate in the Purkinje fibers that penetrate the ventricular myocardium, initiating its contraction from the endocardium toward the epicardium. An organized sequence of impulse generation and conduction is required for the cardiomyocytes to synchronously contract, and, by coordinated chamber contraction, to maintain cardiac output.
Understanding normal cardiac electrophysiology is the foundation for understanding the basic principles of disease mechanisms and antiarrhythmic pharmacotherapy. The following is a brief review.
Cardiac Action Potential
In the normal resting myocardium, transmembrane potential is determined primarily by potassium conductance, and is maintained by the Na+/K+ ATPase. The resting transmembrane potential is stable around −90 mV (the intracellular compartment being more negative relative to the outside of the cell), approaching the potassium equilibrium potential.
An action potential is triggered by either the cardiac pacemaker cells, or by the surrounding cardiomyocytes. This results in a transient increase in Na+ conductance, which in turn initiates an increase in Na+-influx through fast sodium channels. When the threshold potential is reached at around −70 mV, a large enough number of sodium channels have been opened to generate a self-sustaining sodium influx. Above −40 mV, long-opening (L-type) Ca2+-channels open with resultant Ca2+-influx down its concentration gradient. At around 0 mV, fast Na+-channels start to close. This is Phase 0 (early rapid depolarization), peaking at +20 mV (Na+-equilibrium potential).
The increase in Na+-conductance is inactivated. This, with the outward movement of K+ via transient K+-channels results in a brief period of initial repolarization. The transmembrane potential returns from slightly positive to approximately 0 mV. This is Phase 1.
Following the initial repolarization, a constant Ca2+-influx via slow (L-type, “long-opening”) Ca2+-channels, and an increase in K+ conductance via delayed rectifiers maintains the membrane potential just below 0 mV. The electrical countercurrents are balanced. This is Phase 2, the plateau. The phase between the initiation of the upstroke and the end of the plateau is the absolute refractory period, during which the cell is unable to be depolarized regardless of the strength of the stimulus, and corresponds with phase between the beginning of the QRS complex and the beginning of the T wave on the electrocardiogram (ECG).
The next phase of the cardiac action potential is the rapid repolarization. Ca2+-conductance via the L-channels is inactivated, but the K+-channels remain open. Potassium is rapidly shifted out of the cell, and the transmembrane potential rapidly approaches the K+-equilibrium. This is Phase 3. The phase between the initiation of the upstroke and the transition between Phase 2 and 3 is the relative refractory period, during which the cell may be able to be depolarized to allow for a non-propagated stimulus. This corresponds with the early upstroke of the T wave. During phase 3, the cell is able to be depolarized by supranormal stimuli (relative refractory period), resulting in an action potential. This corresponds with the dome of the T wave on the ECG. Membrane hyperpolarization at the end of this phase results in a hyperexcitable period, during which even weak stimuli can trigger an action potential. This brief phase corresponds with the downward slope of the T wave.
During Phase 4, Na+ and Ca2+ channels are closed. There is a constant outflow of potassium ions through inward rectifier channels. This is the resting phase in the contractile cells, or spontaneous diastolic depolarization in the cardiac pacemaker cells.
Pacemaker Cells
Pacemaker cells are found primarily in the dominant and subsidiary rhythm generators (SA node, AV node, His-Purkinje system) of the heart. However, impulses may originate from other sites; for example, cells at the coronary sinus, atrioventricular valves, or cells at the crista terminalis. Abnormally, impulses may originate from around the pulmonary veins. These cells are different from contractile cardiomyocytes in that they share the characteristic to generate spontaneous cardiac action potentials. They exhibit automaticity, have an unstable membrane potential, and have no rapid depolarization phase.
Automaticity means that pacemaker cells are capable of initiating their own action potential without external stimulus. These cells undergo a spontaneous diastolic depolarization and action potential is triggered when threshold potential is reached.
Pacemaker cells undergo spontaneous diastolic depolarization during phase 4 (as opposed to the resting phase during phase 4 in contractile cells), and have no rapid depolarization phase. These cells have fewer inward K rectifier channels than contractile myocytes do, and their transmembrane potential is never lower than −60 mV. Therefore, the fast Na+-channels that need −90 mV to get activated are permanently inactivated in these cells.
Multiple ion currents are responsible for the generation of spontaneous action potentials. The synergy of 3 different currents—(1) the decay of the K+-efflux; (2) an inward depolarizing “funny” mixed Na+-K+ inward current, activated by membrane hyperpolarization, and playing a major role in the spontaneous depolarization of the sinoatrial node; and (3) an inward Ca2+-current in the late phase of the spontaneous diastolic depolarization—determines the rate and shape of action potentials in cardiac pacemaker cells. Physiologically, the dominant pacemaker is the sinoatrial node. When its rate drops below the intrinsic rate of a latent, non-dominant pacemaker—for example, as a result of parasympathetic stimulation or SA nodal disease—the removal of the sinus overdrive leads to “escape-activation” of these non-dominant centers. Junctional rhythm may also occur when the AV junctional pacemaker rate exceeds and suppresses the sinoatrial rate.
Mechanisms of Cardiac Dysrhythmias
Cardiac arrhythmias are commonly defined as abnormalities of the normal sequence of impulse generation and propagation within the myocardium. While benign arrhythmias—for example, premature atrial contractions, isolated premature ventricular contractions, or atrial fibrillation in the absence of structural heart disease—are very common, malignant ventricular arrhythmias (monomorphic or polymorphic ventricular tachycardia [VT], ventricular fibrillation) accounted for an estimated 180,000–250,000 sudden cardiac deaths in the United States.
Underlying provoking factors are, for example, arterial hypoxemia, acidosis or alkalosis, electrolyte abnormalities, ischemia, increased sympathetic activity, atrial or ventricular dilation, or proarrhythmogenic drugs. In some cases, correction of these factors is sufficient to suppress cardiac ectopies. However, when this alone does not control cardiac arrhythmias, and/or hemodynamic compromise ensues, or the presence of a disturbance increases the risk of a life-threatening arrhythmia, administration of antiarrhythmic agents may be warranted.
The main mechanisms of cardiac dysrhythmias have been identified as:
Focal activity due to abnormal impulse generation
This results from:
-
Enhancement or reduction of normal automaticity (such as in sinus tachycardia and sinus bradycardia, accelerated junctional rhythm).
-
Enhanced abnormal automaticity of the Purkinje-fibers, atrial or ventricular myocytes; ie, cells that do not normally display automaticity (for example, atrial tachycardia or accelerated idioventricular rhythm. Purkinje fibers are catecholamine-sensitive, and their activation can be augmented by increased sympathetic tone, for example during myocardial ischemia).
-
Triggered activity. This occurs when early afterdepolarizations and delayed afterdepolarizations initiate spontaneous multiple depolarizations, such as in torsades de pointes, or ventricular arrhythmias in the setting of digitalis toxicity.
Abnormal conduction
Delayed conduction (such as in atrioventricular blocks), or re-entrant mechanisms (for example, SA nodal re-entry, atrial flutter, AV nodal re-entry tachycardia, or ventricular tachycardia). Re-entrant tachycardias can be generated by 3 different mechanisms: reflection, circus movement re-entry, and phase 2 re-entry. Circus movement re-entry due to an anatomical or functional block is a major mechanism and the most commonly described. For a re-entrant circuit to occur, 2 roughly parallel conductive pathways must be connected with conductive tissue, capable of forming an electrical circuit. One of these pathways must have a refractory period substantially longer than that of the other pathway (pathway A). Finally, the pathway with the shorter refractory period (alternate pathway, pathway B) must conduct impulses more slowly than pathway A. Once an extra impulse (for example, premature contraction) encounters these 2 separate pathways of conduction when pathway A is unable to be depolarized due to being in the refractory period but pathway B is capable of depolarization, the impulse will be conducted via pathway B. The signal then travels to the distal end of pathway A to reconnect with it if it is no longer refractory, then it is conducted retrograde to the site where it reconnects with pathway B. Pathway B has a shorter refractory period and therefore it recovers faster: The impulse will travel into pathway B where it will reenter that portion of the circuit, completing the loop. Micro-re-entry occurs with ventricular tachycardia from conduction around scar tissue such as in myocardial infarction (MI). Macro-re-entry occurs via conduction through concealed accessory pathways, such as in Wolff-Parkinson-White syndrome.
Antiarrhythmic Agents: Classification and Mechanism of Action
Pharmacological management of arrhythmias is warranted when treatment of the underlying causes does not break the arrhythmia, the arrhythmia results in hemodynamic compromise, or it increases the risk of development of a life-threatening arrhythmia. The most widely used classification system of antiarrhythmic drugs was proposed by Vaughan Williams. This system classifies the antiarrhythmic agents based on their ability of abolishing an arrhythmia by blocking specific ion currents during the action potential.
Ion-specific channels exist in 3 different stages. During the upstroke phase (phase 0) of the action potential the channels are in the activated state. During the plateau phase of repolarization (phase 2), the inactivated state occurs: During the effective refractory period channels are unresponsive to new or continued stimulus. Ion channels are closed during the resting phase (phase 4).
The effects on the action potential and the effective refractory period of the cardiac action potential determine the clinical effect of antiarrhythmic drugs. Drugs blocking inward sodium ion flow will slow conduction and result in suppression of the maximum upstroke velocity of the cardiac action potential. Potassium channel blockers prolong repolarization by prolonging the duration QTc prolongation. L and T type calcium channels are present in the myocardium, and are targets of calcium channel blockers.
Classification
Class I
Class I antiarrhythmic drugs are fast Na-channel inhibitors. Fast sodium channels are blocked during phase 0 (upstroke) and phase 4 depolarization of the action potential with resultant decreases in the amplitude and rate of the action potential and conduction velocity.
Class IA
for example, quinidine, procainamide, disopyramide. These drugs lengthen both the action potential and the effective refractory period reflecting sodium channel inhibition and lengthening of repolarization reflecting potassium channel blockade. These drugs are considered membrane stabilizers in the treatment of atrial, AV nodal, and ventricular arrhythmias. They may prolong the QRS and QT intervals.
-
Quinidine – Quinidine has mild parasympatholytic and alpha-blocking effects and decreases systemic vascular resistance (SVR).
-
Procainamide – Procainamide is used to suppress premature atrial and ventricular contractions and to prevent precipitation of atrial fibrillation or flutter. It increases refractoriness and can prevent accessory pathway re-entry tachycardias. High serum levels may cause direct myocardial depression and bradycardia requiring temporary pacing or administration of beta-agonists. Procainamide and its active metabolite N-acetylprocainamide may induce QT-prolongation and torsades de pointes. Long-term therapy may cause lupus-like symptoms.
-
Disopyramide – Disopyramide has a vagolytic effect that is dose-dependent and reversible by pyridostigmine. It depresses myocardial contractility and increases SVR, and may thus precipitate or exacerbate congestive heart failure.
Class IB
for example, lidocaine, tocainide, mexiletine. These are less powerful Na-channel blockers. They shorten action potential duration and refractory period in normal ventricular myocytes. Class II drugs have minimal effect on inotropy or SVR. In the ischemic tissue, lidocaine may block adenosine triphosphate-dependent channels, preventing ischemic-mediated shortening of ventricular depolarization. Lidocaine depresses the slope of phase 4 depolarization in Purkinje fibers and increases the fibrillation threshold in ventricular myocytes. Signs of toxicity are frequent with concentrations above 9 mcg/ml.
Class IC
for example, flecainide, propafenone. Class IC drugs are potent sodium channel blockers, indicated for the treatment of ventricular arrhythmias. They markedly decrease the rate of phase 0 depolarization and speed of conduction. They have little effect on the duration of the action potential and the effective refractory period in ventricular muscular cells, but do shorten the duration of the action potentials in the Purkinje fibers. This inhomogeneity on the rate of cardiac repolarization plus the slowing of cardiac conduction may contribute to the prodysrhythmic effects of these drugs particularly in patients with history of myocardial infarction, left ventricular dysfunction, or previous sustained ventricular tachycardia. Class IC drugs, especially flecainide, significantly depress inotropy and prolong the PR and QT intervals.
Class II
Class II drugs are beta-adrenergic receptor blockers. Beta-adrenergic receptor antagonists decrease the rate of spontaneous phase 4 depolarization, important in suppression of ventricular arrhythmias during ischemia and reperfusion. They are effective in the treatment of adrenergically mediated disease states, in which increased phase 4 depolarization, enhanced conduction velocity, and a shorter refractory period all contribute to increased automaticity. Beta-blockers decrease the rate of Vmax of the action potential, prolongs its duration as well as the effective refractory period. Drug-induced slowing of the heart rate with resulting decreases in myocardial oxygen requirements is desirable in patients with coronary artery disease. Beta blockers slow the sinus rate, prolong AV-nodal conduction and enhance refractoriness. They prolong the PR interval on the ECG. Esmolol is used to convert atrial fibrillation with rapid ventricular response to normal sinus rhythm, or to maintain a slow ventricular rate. It also predictably reverses the fibrillation threshold lowering effects of catecholamines.
Class III
Class III drugs are potassium channel blockers; for example, amiodarone, bretylium, sotalol. These drugs prolong cardiac repolarization, action potential duration, and the effective refractory period possibly by interference with sodium and calcium exchange. These effects are beneficial in preventing cardiac dysrhythmias by decreasing the proportion of the cardiac cycle during which myocardial cells are excitable and susceptible to a triggering event. Reentrant tachycardias may be suppressed if the action potential duration becomes longer than the cycle length of the tachycardia circuit.
-
Amiodarone – In addition to class III effects, amiodarone exhibits class I Na+-channel blocking, class II beta blocking, and class IV Ca2+-channel blocking properties. It prolongs repolarization and cardiac action potential, it produces negative chronotropy in nodal tissues and as a Ca2+ and K+-channel blocker, it slows SA-nodal conduction speed and prolongs refractory period. It is an α- and β-receptor antagonist with potent vasodilating and myocardial depressant potential. It may produce sinus bradycardia or heart block, necessitating administration of positive chronotropes or initiation of temporary pacing. Long-term side effects include pulmonary fibrosis, corneal microdeposits, liver cirrhosis, hyperthyroidism, or hypothyroidism.
-
Sotalol – Sotalol is a mixture of isomers that possess similar class III effects, used for the treatment of atrial fibrillation or atrial flutter, as well as to treat ventricular arrhythmias. The L isomer of sotalol acts as a beta antagonist, whereas the D isomer may increase mortality in patients with ventricular dysfunction and recent myocardial infarction. Sotalol lacks intrinsic sympathomimetic activity or membrane-stabilizing properties. The lower incidence of dysrhythmia effects seen with amiodarone and racemic sotalol may be related to the beneficial class II effects.
-
Bretylium is no longer available in the United States for clinical use.
Class IV
Calcium channel blockers: These agents act by inhibiting inward slow inward calcium currents that may contribute to the development of ventricular arrhythmias. These drugs are useful in the treatment of idiopathic ventricular tachycardias. Calcium channel blockers prolong neuromuscular blockade.
Prodysrhythmic Effects
Prodysrhythmia effects of antiarrhythmic agents describe bradydysrhythmias or tachydysrhythmias that represent new dysrhythmias associated with chronic antidysrhythmic drug treatment. Types of dysrhythmias include torsades de pointes, incessant VT, and wide complex ventricular rhythm.
Torsades de pointes (polymorphic VT) is the most common dysrhythmia and is triggered by early after-depolarizations in a setting of a delayed depolarization and increased duration of refractoriness manifesting as prolonged QTc interval on the ECG. Class IA (especially quinidine) and class III drugs prolong QTc by potassium channel blockade and provide the setting for torsades de pointes. Drug-induced torsades is often associated with bradycardia, because the QTc interval is longer at slower heart rates. Exacerbating factors such as hypokalemia, hypomagnesemia, poor LV function, and concomitant administration of other QT-prolonging drugs are important predisposing factors in the development of torsades de pointes.
Incessant ventricular tachycardia may be precipitated by cardiac antidysrhythmic drugs that slow conduction of cardiac impulses (class IA and class IC) sufficiently to create a continuous ventricular reentry circuit. Incessant ventricular tachycardia is more likely to occur with high doses of class IC drugs and in patients with prior history of sustained ventricular tachycardia and poor LV function. Ventricular tachycardia due to this mechanism is generally slower because of the drug effect, but may be resistant to drugs or electrical therapy.
Wide complex ventricular rhythm is usually associated with class IC drugs in the setting of structural heart disease with excessive plasma concentrations or abrupt changes in the dose. Wide complex rhythm is thought to reflect a reentrant tachycardia and easily degenerates to ventricular fibrillation.
11.2.3 Antianginal Drugs: Coronary Vasodilators and Cardioinhibitory Drugs
Under normal physiological conditions oxygen delivery (DO2, the amount of oxygen delivered to the cells) is adequate to meet cellular oxygen demand (VO2, the amount of oxygen extracted from arterial blood) and maintain aerobic metabolism. The ratio of oxygen consumption to the oxygen available to tissues is the oxygen extraction ratio, which varies for different organs. During periods of increased workload and increased cellular demand, and/or decreased supply, aerobic metabolism can still be maintained independently of blood flow by increasing O2 extraction. Critical O2 delivery is the point at which the extraction ratio is maximized, and any further incongruence between demand and supply will lead to tissue hypoxia and subsequent activation of anaerobic metabolic pathways. When myocardial oxygen consumption exceeds the reserve in coronary blood supply to meet a given O2 demand, ischemia is precipitated. The heart’s high, 60–70% O2 extraction ratio (compared to 25–30% for the rest of the body) can make it susceptible to even short periods of ischemia.
The major determinants of myocardial O2 supply are coronary blood flow and arterial O2 content. Coronary blood flow is further determined by the patency of the coronaries, coronary perfusion pressure, and coronary vascular resistance. The 3 major determinants of myocardial O2 demand are heart rate, inotropic state, and wall tension (which is the function of intracavitary pressure, radius, and wall thickness, as well as preload and afterload). Antianginal pharmacotherapy is indicated when the supply/demand imbalance results in ischemia. In accordance with the 2014 American College of Cardiology/American Heart Association (ACC/AHA) Guideline for the Management of Patients with Non-ST-Elevation Acute Coronary Syndromes, standard medical therapy includes the use of beta blockers, nitroglycerin, calcium channel blockers, analgesics, and cholesterol management. This section will focus on role of nitroglycerin, beta-blockers and calcium channel blockers in the management of angina pectoris.
Coronary Vasodilators
Nitroglycerin
Nitroglycerin (NTG) is an endothelium-independent smooth muscle relaxant that acts predominantly on venous capacitance vessels and large epicardial coronary arteries, maximizing blood flow to the subendocardial areas. Peripheral venodilatory effects are prominent even at low doses and are not dose-dependent, whereas dilation of peripheral conductance and resistance vessels occur at higher doses, and further increases in dose result in more pronounced vasodilation. Nitroglycerin reduces myocardial O2 demand and increases oxygen supply by reducing preload and decreasing ventricular dimension and wall tension, dilating normal and atherosclerotic coronary arteries, and enhancing collateral circulation. The degree to which coronaries are able to dilate is dependent on their baseline vascular tone. Nitroglycerin produces a dose-dependent systemic and pulmonary arterial vasodilatory effect, and predictably decreases cardiac filling pressures. Its use is indicated for initial treatment of nearly all types of myocardial ischemia, as well as for managing hypertension and heart failure. Elimination half-life of nitroglycerin is approximately 1.5 min.
Mechanism of Action
NTG enters the smooth muscle cells and generates nitric oxide through a glutathione-dependent pathway, which stimulates cyclic GMP production and causes peripheral vasodilation via a sequence of protein phosphorylation and dephosphorylation within the smooth muscle. Nitric oxide production via guanylate cyclase stimulation and cGMP production is a sulfhydryl (SH)-group dependent process. Depletion of SH-groups by prolonged exposure leads to dose- and duration-dependent tolerance, which may manifest within the first 24 hours of treatment. Restoring SH-supplies with reducing agents (for example, N-acetylcysteine) does not reliably reverse nitrate tolerance, therefore a drug-free interval of 12–24 hours is recommended to maintain drug efficacy. Rebound myocardial ischemia may occur during drug-free intervals.
Common Clinical Use
Nitroglycerin is available in intravenous (IV), sublingual, or topical formulations. A typical IV dose to treat acute ischemia is 50–10 mcg. For a continuous infusion, the dose range is 0.1–7 mcg/kg/min.
Adverse Effects
Nitroglycerin at low doses causes predominantly splanchnic venodilation that results in venous pooling, decreased cardiac filling, and decreased biventricular end-diastolic volume and pressure. Excessive decreases in diastolic blood pressure may decrease coronary blood flow and trigger reflex tachycardia and increased contractility mediated by the baroreceptor reflex. The combination of decreased coronary blood flow and increased myocardial O2 demand may provoke angina pectoris. With administration of intravenous phenylephrine, adequate coronary perfusion pressure can be maintained.
In the lungs, nitroglycerin inhibits hypoxic pulmonary vasoconstriction and worsens hypoxia and gas exchange. It has been shown to have platelet-inhibitory effects, the clinical significance of which has not been elucidated.
Although an uncommon complication of nitroglycerin therapy, the nitrite metabolite of NTG is capable of oxidizing the ferrous (Fe2+) ion in the hemoglobin into the ferric (Fe3+) state, producing methemoglobin. Ferric (oxidated) iron is unable to carry O2 effectively, and in sufficiently high concentrations (> 40%) it can impair tissue oxygenation. Treatment of methemoglobinemia is methylene blue, to facilitate the conversion of methemoglobin to hemoglobin. High doses of NTG is more likely to produce methemoglobinemia in patients with hepatic dysfunction.
The 2014 ACC/AHA guidelines recommend that nitrates should not be administered to patients with non-ST-elevation-acute coronary syndrome (ACS) who recently received a phosphodiesterase inhibitor (class III, level of evidence: B). Nitrates should not be administered to patients with signs of hypovolemia or hypotension, and it should be used with caution in patients with right ventricular infarction.
Cardioinhibitory Drugs
Beta-Adrenergic Blockers
As discussed in the previous section, beta-blockers are class II antiarrhythmics with a multitude of favorable properties utilized in the treatment of cardiac ischemia. Their negative inotropic, negative chronotropic, and antihypertensive properties allow for reduction in O2 consumption and an increase in blood supply during diastole. Beta blockers slow spontaneous diastolic depolarization and shorten the duration of cardiac action potentials. Ventricular fibrillation threshold is increased. Beta blockers reduce myocardial infarct size. In the absence of contraindications (cardiogenic shock, decompensated heart failure, severe sinus bradycardia, second- or third-degree atrioventricular block, or active bronchospasm), beta blockers should be administered early in the treatment of myocardial ischemia. While early administration has not been shown to improve short-term survival, beta blockers decrease reinfarction and the frequency of ventricular dysrhythmias, and their use has been associated with mortality benefit after myocardial infarction. Perioperative beta blockers should be continued in patients already receiving beta blockers, according to class 1 indications for perioperative beta blockade from the 2014 ACC/AHA recommendations; patients at high perioperative risk for adverse cardiac events should be started on beta blockers preoperatively, and continued up to 30 days postoperatively. In these patients, it is reasonable to titrate beta blockers to heart rate and blood pressure.
Mechanism of Action
Beta blockers are competitive, reversible inhibitors of beta adrenergic receptors. Beta adrenergic receptors are G-protein coupled receptors. Stimulation by their agonists activates adenylate cyclase to produce cyclic AMP. Cyclic AMP in turn activates protein kinases pathways with subsequent phosphorylation of L-type calcium channels and troponin C, the net effect being enhanced inotropy, positive chronotropy and dromotropy. These responses are all blunted by receptor occupancy by beta antagonists.
-
Propranolol – Propranolol is a non-selective β(beta)-1 and β(beta)-2 receptor blocker with no alpha-receptor activity. As the most soluble beta blocker, its use is associated with the highest frequency of central nervous system side effects. It is well absorbed when taken by mouth. For a comparable effect, higher oral than intravenous doses are required due to its very high (90%) hepatic first-pass metabolism. Its active metabolite does not add to the primary clinical effect due to its short half-life. Propranolol decreases cardiac output by decreasing the heart rate and reducing myocardial contractility, effects that are especially prominent in sympathetically driven disease states. Owing to its β(beta)-2 receptor antagonism, propranolol may increase systemic vascular and coronary vascular resistance. Increased airway resistance may be evoked by propranolol in asthmatic patients. Renal and hepatic blood flow is reduced with the administration of propranolol.
-
Metoprolol – Metoprolol was the first β(beta)-1 selective receptor antagonist used in clinical practice to prevent increased chronotropy and inotropy in response to sympathetic stimulation. Its receptor selectivity is dose related. Metoprolol is lipid-soluble, it diffuses more readily into ischemic regions than hydrophilic drugs. Fifty percent of the drug administered is metabolized during first-pass hepatic metabolism. At the intravenous dose of 0.2 mg/kg, maximum beta receptor blockade is achieved.
-
Esmolol – Esmolol is a short-acting cardioselective agent metabolized rapidly by red blood cell esterases. It is primarily a β-1 blocking agent, producing significant decreases in heart rate and contractility. It lacks the ability to block peripheral vascular β-2 receptors, therefore decreases in blood pressure and cardiac index is more pronounced due to unopposed peripheral vasodilation. Esmolol has been safely used in patients with reactive airway disease.
-
Sotalol – Sotalol is a class III antiarrhythmic agent with both β-receptor and K+-channel antagonistic effects. A relatively recent Cochrane database review found significant reductions in incidence of postoperative atrial fibrillation in the cardiac surgical population. Despite the conclusions of this review, the use of sotalol in the post-cardiac surgical population remains limited, owing to sotalol’s undesired side effects, such as hypotension, bradycardia, QT-prolongation, and inducing torsade-type ventricular arrhythmias.
Calcium Channel Blockers
Calcium channel blockers (CCBs) comprise a diverse group of agents that selectively inhibit calcium influx into myocardial and vascular smooth muscle cells. Dihydropyridines (such as nifedipine, nicardipine, nimodipine, amlodipine, felodipine, isradipine) exert their effect on the peripheral arteriolar beds (nimodipine favors cerebral vessels), and produce marked peripheral vasodilation with little direct effect on heart rate, AV conduction, and inotropy. They may elicit reflex sympathetic activation via the baroreceptor reflex. Non-dihydropyridines (phenylalkylamines, for example, verapamil; and benzothiazepines, for example, diltiazem), on the other hand, block AV nodal calcium channels, and have significant negative inotropic, chronotropic, and dromotropic effects. Their main anti-ischemic effects are due to their ability to reduce myocardial O2 consumption by depressing contractility, decreasing heart rate and systemic afterload, and increasing O2 supply by coronary and collateral vasodilation. Calcium channel blockers are preferred in vasospastic (variant) angina, as beta-blockers may provoke or aggravate ischemia in some patients. All calcium channel blockers are effective in the treatment and prevention of coronary vasospasm. They may reduce reinfarctions and long-term events in hypertensive patients with acute myocardial infarction.
Calcium Channels
Calcium channels display selectivity to calcium ions, and are present among others in myocardial, smooth muscle, skeletal muscle, and neural tissues, as well as in membranes of subcellular organelles, such as the mitochondria or the sarcoplasmic reticulum. Calcium channel blockers bind to voltage-gated transient (T), long (L) type channels in the myocardium and smooth muscle, or to neural (N) type channels, rendering them inactive. T-type calcium channels are activated at low voltages, play a major role in the phase 0 depolarization, and are not affected by calcium channel blockers. L-type channels are activated at higher voltages, playing a role in the phase 2 plateau of the cardiac action potential. These are the Ca2+-channels that are able to be blocked by calcium antagonists.
Dihydropyridines: Smooth-Muscle-Selective Vasodilators
Dihydropyridines prevent calcium entry into the vascular smooth muscle cell by extracellular modulation of the L-type channels. The primary target of dihydropyridines is the peripheral arteriolar bed except for nimodipine, which favors cerebral vessels. Reflex tachycardia may be elicited with their use. Examples include nifedipine, nicardipine, nimodipine, amlodipine, and felodipine. The predominant action of these agents is decreasing systemic, coronary, and cerebrovascular vasomotor tone. Dihydropyridine calcium channel blockers, with the exception of the primarily antianginal nifedipine, will be discussed later.
-
Nifedipine – Nifedipine was the first dihydropyridine calcium channel blocker in clinical use for its coronary and peripheral vasodilator properties. It has greater coronary and peripheral vasodilatory properties than verapamil with negligible effects on venous capacitance vessels. It has little or no effect on cardiac impulse generation and on SA and AV nodal conduction. Peripheral vasodilation and the resultant decrease of systemic blood pressure activate baroreceptors, leading to reflex sympathetic nervous system activity manifesting as tachycardia. In the absence of concomitant beta-receptor blockade, it may increase the risk of myocardial infarct or recurrent angina. Excessive peripheral vasodilation can be antagonized with phenylephrine. It may be combined with beta blockers without increasing the risk of AV-block. Nifedipine is used in angina pectoris due to coronary artery vasospasm. No IV preparation is available due to its extreme instability when exposed to light. Its abrupt discontinuation has been associated with coronary artery vasospasm.
Non-Dihydropyridines: Antiarrhythmics
Phenylalkilamines
Phenylalkilamines bind to the intracellular portion of the L-type calcium channel when it is in the open state, and occlude the channel.
-
Verapamil – Verapamil is a synthetic derivative of papaverine. It is supplied as a racemic mixture, in which the D-isomer lacks Ca2+-channel blocking properties and acts as a fast Na+-channel blocker, accounting for local anesthetic effects, and the L-isomer is specific for slow Ca2+-channels. The predominance of this action accounts for the classification of this drug as a calcium channel blocker. Verapamil decreases the heart rate by depressing sinoatrial and AV-nodal activity (hence its utility in the treatment of supraventricular arrhythmias), lowers systemic blood pressure due to myocardial depression and peripheral vasodilation, and produces moderate coronary artery dilation (preferred in essential hypertension and vasospastic angina). Its negative inotropy is more pronounced in patients who already have a depressed left ventricular function, therefore verapamil should be avoided in symptomatic heart failure, severe bradycardia, sinus node dysfunction, and AV nodal block. These effects of verapamil may be enhanced with concomitant β(beta)-blockade. In the presence of drug-induced heart block, isoproterenol may be useful to increase heart rate. Verapamil may also precipitate dysrhythmias in patients with Wolff-Parkinson-White (WPW) syndrome, and has proven effective in the treatment of hypertrophic cardiomyopathy with or without left ventricular outflow tract (LVOT) obstruction. Verapamil may be useful in the treatment of premature labor, as well as fetal and maternal tachydysrhythmias. It may decrease uterine blood flow, and should be administered with caution to parturients with impaired uteroplacental perfusion.
Stay updated, free articles. Join our Telegram channel
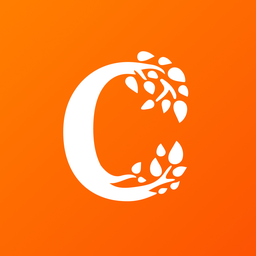
Full access? Get Clinical Tree
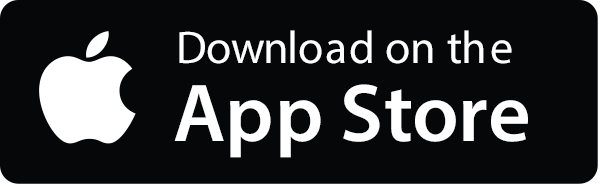
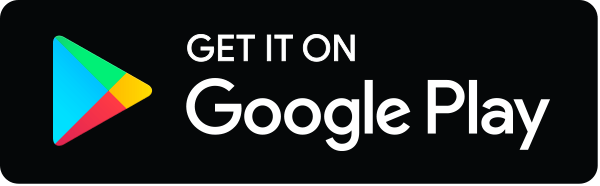