Abstract
Resuscitation maneuvers improve oxygen delivery, optimize tissue uptake of oxygen, and preserve the metabolic rate of oxygen. This chapter reviews the cardinal tenets of oxygenation, the evidence-based practices of resuscitating the critically ill patient, and the physiology of cardiopulmonary resuscitation (CPR). Our understanding of CPR physiology continues to grow, but morbidity and mortality after cardiac arrest remain poor. Optimizing circulation during CPR and avoiding common errors encountered are initial steps toward improving outcomes. Advances and experience with new technology, including automated devices and extracorporeal life support, are actively being pursued.
Keywords
critical oxygen delivery, early goal-directed therapy, cardiac pump theory, thoracic pump theory, extracorporeal cardiopulmonary resuscitation
Chapter Outline
Oxygen Delivery, Consumption, and the Margin of Error
Compensated Hypovolemia and Supply-Dependent Oxygen Consumption
Ventilation and Blood Flow During CPR
Optimizing Chest Compression/Decompression
Automated Chest Compression Devices
The objective of cardiopulmonary resuscitation CPR) in the critically ill patient is optimization of both oxygen delivery (D o 2 ) and aerobic metabolism. Shock is defined as dysoxia, or an abnormality in tissue oxygenation. The variables altering the clinical state of shock include D o 2 , tissue uptake of oxygen (V̇ o 2 ), and the tissue metabolic rate of oxygen (MR o 2 ). The various shock states are defined by causes of decreased Do 2 . Resuscitation maneuvers improve Do 2 , optimize tissue uptake of oxygen, and preserve metabolic rate of oxygen. This chapter reviews the cardinal tenets of oxygenation, the physiology of CPR, and the scientific basis of practices of resuscitating the critically ill patient.
Historical Perspective
Peter Safar is credited with the first published account of expired gas ventilation via mouth-to-mouth or mouth-to-airway techniques using a novel device of two fused oral airways in 1957. Closed chest compression in humans was famously first described in the medical literature by Kouwenhoven and colleagues in 1960. However, origins of CPR are evident in 18th-century descriptions the management of drowning victims, as well as prior Biblical descriptions of Elisha who “went up and lay on the child and put his mouth on his mouth…and the flesh of the child became warm.” In the 1960s the combination of chest compressions and mouth-to-mouth ventilation was popularized as “cardiopulmonary resuscitation” or CPR. It was at this time that the American Heart Association (AHA) was formed and took up the task of teaching physicians, and later the general public, to perform CPR. An ad hoc committee wrote the first guidelines for CPR established by the National Academy of Sciences of the National Research Council in 1966. Over the past 50 years, a breadth of research and several revisions to the CPR guidelines were made; however, survival rates for both out-of-hospital and in-hospital survival for in-hospital cardiac arrest and CPR are 15% and 22%, respectively. Neurologic deficits occur in 30% to 50% of patients after out-of-hospital cardiac arrest.
Oxygen Delivery, Consumption, and the Margin of Error
Normal homeostatic mechanisms ensure greater Do 2 than the total tissue uptake of oxygen (D o 2 > V̇ o 2 ; see Chapter 24 ). This “margin of error” maintains aerobic metabolism during extreme situations, when Do 2 is diminished, or when tissue oxygen uptake increases. Do 2 is the process by which oxygen is transported from the pulmonary system to the systemic capillary beds (expressed as milliliters per minute per meter squared), and is expressed as the product of the cardiac index (CI) and arterial blood oxygen content (Ca o 2 ): D o 2 = CI * Ca o 2 . The cardiac index is the cardiac output (Q) normalized for body surface area (BSA): CI = Q/BSA. Normal values of cardiac output and cardiac index in an average size adult are 4.0 to 8.0 L/min and 2.5 to 4.0 L/min per meter squared, respectively. The content of Ca o 2 depends on the volume of oxygen bound to hemoglobin (Hbg) and of free oxygen dissolved in blood (Ca o 2 = 1.39 * Hgb * Sa o 2 + 0.003 * Pa o 2 ), where Pa o 2 is the partial pressure of alveolar oxygen. Sa o 2 represents the oxygen saturation, Pa o 2 the arterial oxygen tension (in millimeters of mercury), and 0.003 the plasma solubility coefficient of oxygen ( Table 28.1 ). The vast majority of oxygen is bound to hemoglobin such that even at very high oxygen tensions the additional dissolved oxygen contributes little to overall Ca o 2 .
Cardiac index (CI) | CI = Q/BSA |
Arterial oxygen content (Ca o 2 ) | Ca o 2 = 1.39 * Hgb * Sa o 2 + 0.003 * Pa o 2 |
Oxygen delivery (D o 2 ) | D o 2 = CI * Ca o 2 |
Oxygen consumption (V̇ o 2 ; Fick principle) | V̇ o 2 = Q*(Ca o 2 − Cv o 2 ) |
Mixed venous oxygenation (Scv o 2 ; Fick principle) | Scv o 2. = Sa o 2 − [V̇ o 2 /(Hgb * 1.36 * Q)] |
Oxygen extraction ratio (O 2 ER) | O 2 ER = V̇ o 2 /D o 2 |
Drug | Indication | IV/IO Dosage |
---|---|---|
Adenosine | Tachycardia | 6 mg, may administer second dose of 12 mg |
Amiodarone | Tachycardia | 150 mg over 10 min |
Amiodarone | VT/VF | 300 mg, repeat 150 mg |
Atropine | Bradycardia | 0.5 mg, repeat 3–5 min up to 3 mg |
Diltiazem | Tachycardia | 15–20 mg over 2 mins, then 20– 25 mg after 15 mins |
Dopamine i nfusion | Bradycardia | 2–10 µg/kg/min |
Epinephrine | VT/VF/asystole/PEA | 1 mg, repeat 3–5 min |
Epinephrine i nfusion | Bradycardia | 2–10 µg/kg/min |
Lidocaine | VT/VF | 1–1.5 mg/kg, then 0.5–0.75 mg/kg every 5 min |
Magnesium s ulfate | Torsade de pointes | 2 g |
Metoprolol | Tachycardia | 5 mg, repeat 2–5 min up to 20 mg |
Procainamide i nfusion | Tachycardia | 20–50 mg/min |
Sotalol | Tachycardia | 100 mg (1.5 mg/kg) over 5 min |
Verapamil | Tachycardia | 2.5–5 mg, then 5–10 mg every 15–30 min |
The oxygen uptake from systemic capillaries (V̇ o 2 ) represents oxygen consumption, as oxygen is not stored in tissue. The Fick principle derives oxygen consumption from the difference in oxygen content between arterial and venous blood, V̇ o 2 = Q * (Ca o 2 − Cv o 2 ). Normal oxygen consumption in a resting adult is 110 to 160 mL/min per meter squared; V̇ o 2 less than 100 mL/min meter squared indicates impaired tissue oxygenation. The oxygen extraction ratio (O 2 ER) is the ratio of oxygen consumption to Do 2 (O 2 ER = V̇ o 2 /D o 2 ), and reflects tissue avidity for oxygen. Normal, global O 2 ER is 0.2 to 0.3, so only 20% to 30% of delivered oxygen is used. The O 2 ER varies for different tissues, with renal O 2 ER less than 0.15 and cardiac O 2 ER greater than 0.6.
Mixed venous oxygen saturation reflects the state of global oxygen utilization. Oxygen that is not extracted returns to the mixed venous circulation. Assuming an Sa o 2 of 100%, normal mixed venous saturation (Scv o 2 ) of 70% implies 30% extraction of the oxygen delivered. Rearrangement of the Fick equation yields Scv o 2 = Sa o 2 − V̇ o 2 /(Hgb * 1.36 * Q). Normal Scv o 2 sampled from the pulmonary artery is 65% to 75%, implying an oxygen extraction of ~25% to 35%. Scv o 2 is inversely proportional to oxygen consumption and directly proportional to arterial saturation, hemoglobin concentration, and cardiac output. Therefore maneuvers to improve Scv o 2 aim to increase arterial oxygen content and delivery.
Compensated Hypovolemia and Supply-Dependent Oxygen Consumption
In shock, Do 2 is reduced and in response vital organ beds become more oxygen avid. The O 2 ER increases to maintain aerobic metabolism. Extraction can rise from 20% to 30% to a maximal extraction of 80%. A diminished margin of error (normal D o 2 > V̇ o 2 ) of Do 2 is compensated by a greater O 2 ER. “Compensated hypovolemia” describes the response to decreased Do 2 in which increased O 2 ER allows maintenance of V̇ o 2 . When D o 2 diminishes to a critical point, maximal extraction has been reached, aerobic metabolism is no longer supported, V̇ o 2 is wholly dependent of Do 2 and cellular energy production is limited by the supply of oxygen. This threshold of D o 2 marks tissue dysoxia and the shock state ( Fig. 28.1 ).

The critically ill patient often survives below the critical D o 2 and organ perfusion is entirely supply dependent. This can be due to blood flow redistribution or direct tissue injury, which prevents changes in the O 2 ER. A static O 2 ER hinders a patient’s ability to maintain aerobic metabolism in the face of decreased delivery. This supply dependence underscores the importance of Do 2 during shock and demonstrates the physiologic basis of goal-directed therapy.
Early Goal-Directed Therapy
Evidence supports maximizing Do 2 in high-risk surgical patients to replenish tissue oxygen and prevent organ dysfunction. Resuscitation to supranormal D o 2 after severe trauma improves survival and decreases ventilator days, mean organ failures per patient, and total intensive care unit days. Similar management of septic shock reduces overall hospital mortality; CI and D o 2 are augmented by volume expansion, blood transfusion, mechanical ventilation with a high fraction of inspired oxygen (F io 2 ), and inotropic drugs. In this 1990 cohort, these measures reduced septic shock mortality from 70% to 80% to 59%. The dynamic nature of septic shock is described as an ebb and flow, shifting from hyperdynamic physiology to an end-stage hypodynamic state. The mortality benefit from these early studies is attributed to the perpetuation of a hyperdynamic state.
The basis of goal-directed therapy is the benefit of maximizing parameters of Do 2 . The natural history of septic shock involves an imbalance of Do 2 and oxygen demand, leading eventually to global dysoxia and death. This progression hinges on the critical “golden hour” during which the pathophysiology transitions to multiorgan failure. In a seminal 2000 trial, Rivers and colleagues recommended expeditious, protocolized intervention during this golden hour. Interventions aim to augment Do 2 in the first 6 hours after onset of septic shock by increasing central venous pressure with intravascular volume expansion (central venous pressure goal 8–12 mm Hg), increasing mean arterial pressure (MAP) with vasoactive agents (mean arterial pressure goal 65–90 mm Hg), and maximizing Scv o 2 with red cell transfusion and inotropic support (Scv o 2 > 70%). The Surviving Sepsis Campaign, an international consortium tasked to recommend best practices for sepsis management, endorsed early goal-directed therapy as first-line treatment. This “6-hour bundle” was disseminated internationally as the standard of care for sepsis management by the Surviving Sepsis Campaign as well as the U.S. National Quality Forum and Centers for Medicare and Medicaid Services. This streamlined approach to sepsis management consists of risk stratification to identify the transition from systemic inflammatory response syndrome to severe disease, source control and antibiotic therapy, and hemodynamic optimization ( Fig. 28.2 ).

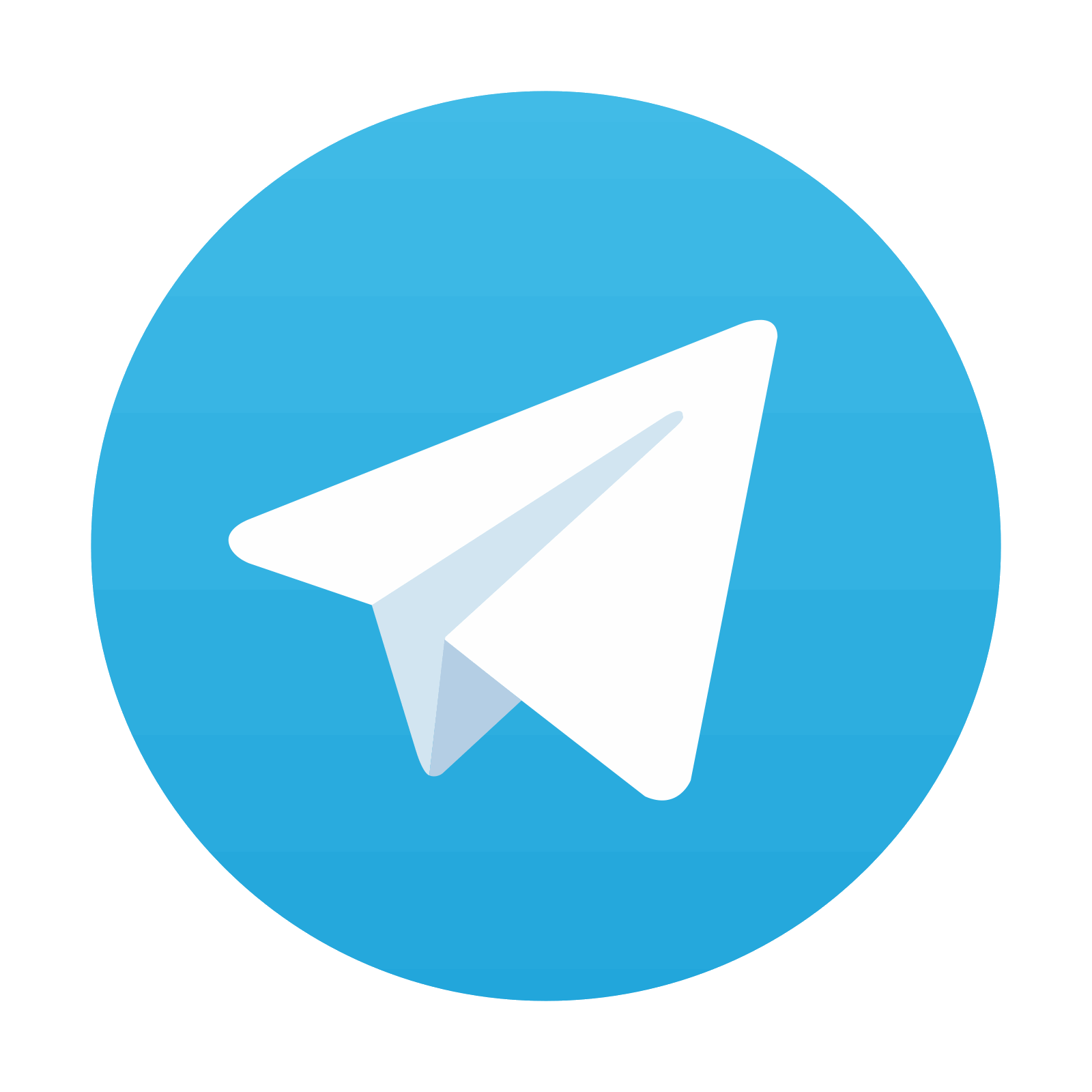
Stay updated, free articles. Join our Telegram channel
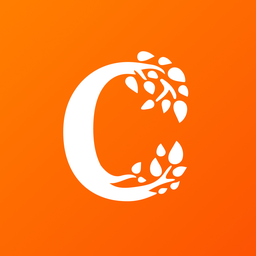
Full access? Get Clinical Tree
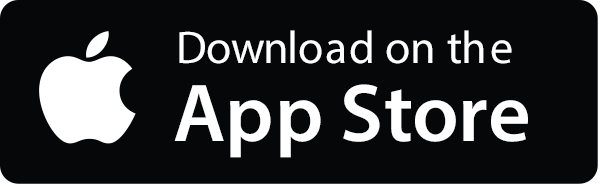
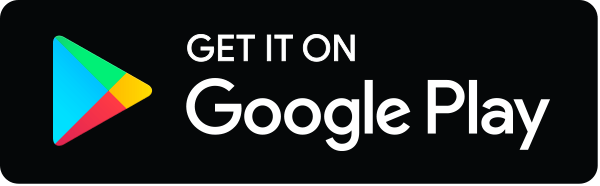
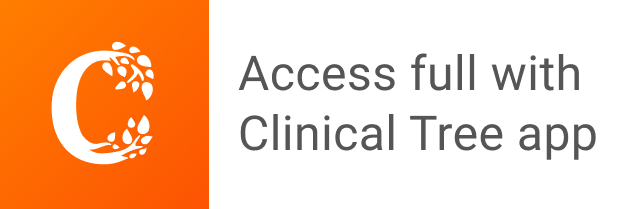