Key Points
- ▪
The cardiac cycle is the sequence of electrical and mechanical events during a single heartbeat.
- ▪
Cardiac output is determined by the heart rate, myocardial contractility, and preload and afterload.
- ▪
The majority of cardiomyocytes consist of myofibrils, which are rod-like bundles that form the contractile elements within the cardiomyocyte.
- ▪
The basic working unit of contraction is the sarcomere.
- ▪
Gap junctions are responsible for the electrical coupling of small molecules between cells.
- ▪
Action potentials have four phases in the heart.
- ▪
The key player in cardiac excitation-contraction coupling is the ubiquitous second messenger calcium.
- ▪
Calcium-induced sparks are spatially and temporally patterned activations of localized calcium release that are important for excitation-contraction coupling and regulation of automaticity and contractility.
- ▪
β-Adrenoreceptors stimulate chronotropy, inotropy, lusitropy, and dromotropy.
- ▪
Hormones with cardiac action can be synthesized and secreted by cardiomyocytes or produced by other tissues and delivered to the heart.
- ▪
Cardiac reflexes are fast-acting reflex loops between the heart and central nervous system that contribute to the regulation of cardiac function and the maintenance of physiologic homeostasis.
Acknowledgment
The editors, publisher, and Dr. Lena Sun would like to thank Drs. Johanna Schwarzenberger and Radhika Dinavahi for their contribution to this chapter in the prior edition of this work. It has served as the foundation for the current chapter.
“To err, to be deceived, is human.” This was William Harvey’s gentle repudiation to fellow physicians in his 1628 “Exercitatio Anatomica de Motu Cordis et Sanguinis in Animalibus,” in which he advanced the concepts of circulation with the heart as the central pump, a major break from the centuries-old anatomic teaching of Galen. Modern cardiac physiology includes this as well as concepts of cellular and molecular biology of the cardiomyocyte and regulation of cardiac function by neural and humoral factors. This chapter focuses on the physiology of the heart, beginning with the intact heart and advancing to cellular cardiac physiology. Finally, the various factors that regulate cardiac function are briefly discussed.
The basic anatomy of the heart consists of two atria and two ventricles that provide two separate circulations in series. The pulmonary circulation, a low-resistance and high-capacitance vascular bed, receives output from the right side of the heart, and its chief function is bidirectional gas exchange. The left side of the heart provides output for the systemic circulation. It functions to deliver oxygen (O 2 ) and nutrients and to remove carbon dioxide (CO 2 ) and metabolites from various tissue beds.
Physiology of the Intact Heart
Understanding the mechanical performance of the intact heart begins with the knowledge of the phases of the cardiac cycle and the determinants of ventricular function.
Cardiac Cycle
The cardiac cycle is the sequence of electrical and mechanical events during a single heartbeat. Fig. 14.1 illustrates the electrical events of a single cardiac cycle represented by the electrocardiogram (ECG) with corresponding mechanical events. Left atrial and left ventricular pressures are shown correlated in time with aortic flow and ventricular volume.

Intrinsic to the specialized cardiac pacemaker tissues is automaticity and rhythmicity. The cardiac cycle begins at the sinoatrial (SA) node with the initiation of the heartbeat. Because the SA node can generate impulses at the greatest frequency, it is the natural pacemaker.
Electrical Events and the Electrocardiogram
Electrical events of the pacemaker and the specialized conduction system are represented by the ECG at the body surface. The ECG is the result of differences in electrical potential generated by the heart at sites of the surface recording. The action potential initiated at the SA node is propagated to both atria by specialized conduction tissue that leads to atrial systole (contraction) and the P wave of the ECG. At the junction of the interatrial and interventricular septa, specialized atrial conduction tissue converges at the atrioventricular (AV) node, which is distally connected to the His bundle. The AV node is an area of relatively slow conduction, and a delay between atrial and ventricular contraction normally occurs at this locus. The PR interval represents the delay between atrial and ventricular contraction at the level of the AV node. From the distal His bundle, an electrical impulse is propagated through large left and right bundle branches and finally to the Purkinje system fibers, which are the smallest branches of the specialized conduction system. Finally, electrical signals are transmitted from the Purkinje system to individual ventricular cardiomyocytes. The spread of depolarization to the ventricular myocardium is exhibited as the QRS complex on the ECG. Depolarization is followed by ventricular repolarization and the appearance of the T wave on the ECG.
Mechanical Events
The mechanical events of a cardiac cycle begin with the return of blood to the right and left atria from the systemic and pulmonary circulation, respectively. As blood accumulates in the atria, atrial pressure increases until it exceeds the pressure within the ventricle, and the AV valve opens. Blood passively flows first into the ventricular chambers, and such flow accounts for approximately 75% of the total ventricular filling. The remainder of the blood flow is mediated by active atrial contraction or systole, known as the atrial “kick.” The onset of atrial systole coincides with the depolarization of the SA node and the P wave. While the ventricles fill, the AV valves are displaced upward and ventricular contraction (systole) begins with closure of the tricuspid and mitral valves, which corresponds to the end of the R wave on the ECG. The first part of ventricular systole is known as isovolumic (or isometric) contraction. The electrical impulse traverses the AV region and passes through the right and left bundle branches into the Purkinje fibers, which leads to contraction of the ventricular myocardium and a progressive increase in intraventricular pressure. When intraventricular pressure exceeds pulmonary artery and aortic pressure, the pulmonic and aortic valves open and ventricular ejection occurs, which is the second part of ventricular systole.
Ventricular ejection is divided into the rapid ejection phase and the reduced ejection phase. During the rapid ejection phase, forward flow is maximal, and pulmonary artery and aortic pressure is maximally developed. In the reduced ejection phase, flow and great artery pressures taper with progression of systole. Pressures in both ventricular chambers decrease as blood is ejected from the heart, and ventricular diastole begins with closure of the pulmonic and aortic valves. The initial period of ventricular diastole consists of the isovolumic relaxation phase. This phase is concomitant with repolarization of the ventricular myocardium and corresponds to the end of the T wave on the ECG. The final portion of ventricular diastole involves a rapid decrease in intraventricular pressure until it decreases to less than that of the right and left atria, at which point the AV valve reopens, ventricular filling occurs, and the cycle repeats itself.
Ventricular Structure and Function
Ventricular Structure
The specific architectural order of the cardiac muscles provides the basis for the heart to function as a pump. The ellipsoid shape of the left ventricle (LV) is a result of the laminar layering of spiraling bundles of cardiac muscles ( Fig. 14.2 ). The orientation of the muscle bundle is longitudinal in the subepicardial myocardium and circumferential in the middle segment and again becomes longitudinal in the subendocardial myocardium. Because of the ellipsoid shape of the LV, regional differences in wall thickness result in corresponding variations in the cross-sectional radius of the left ventricular chamber. These regional differences may serve to accommodate the variable loading conditions of the LV. In addition, such anatomy allows the LV to eject blood in a corkscrew-type motion beginning from the base and ending at the apex. The architecturally complex structure of the LV thus allows maximal shortening of myocytes, which results in increased wall thickness and the generation of force during systole. Moreover, release of the twisted LV may provide a suction mechanism for filling of the LV during diastole. The left ventricular free wall and the septum have similar muscle bundle architecture. As a result, the septum moves inward during systole in a normal heart. Regional wall thickness is a commonly used index of myocardial performance that can be clinically assessed, such as by echocardiography or magnetic resonance imaging.

Unlike the LV, which needs to pump against the higher-pressure systemic circulation, the right ventricle (RV) pumps against a much lower pressure circuit in the pulmonary circulation. Consequently, wall thickness is considerably less in the RV. In contrast to the ellipsoidal form of the LV, the RV is crescent shaped; as a result, the mechanics of right ventricular contraction are more complex. Inflow and outflow contraction is not simultaneous, and much of the contractile force seems to be recruited from interventricular forces of the LV-based septum.
An intricate matrix of collagen fibers forms a scaffold of support for the heart and adjacent vessels. This matrix provides enough strength to resist tensile stretch. The collagen fibers are made up of mostly thick collagen type I fiber, which cross-links with the thin collagen type III fiber, the other major type of collagen. Elastic fibers that contain elastin are in close proximity to the collagen fibers and account for the elasticity of the myocardium.
Ventricular Function
The heart provides the driving force for delivering blood throughout the cardiovascular system to supply nutrients and to remove metabolic waste. Because of the anatomic complexity of the RV, the traditional description of systolic function is usually limited to the LV. Systolic performance of the heart is dependent on loading conditions and contractility. Preload and afterload are two interdependent factors extrinsic to the heart that govern cardiac performance.
Diastole is ventricular relaxation, and it occurs in four distinct phases: (1) isovolumic relaxation; (2) the rapid filling phase (i.e., the LV chamber filling at variable left ventricular pressure); (3) slow filling, or diastasis; and (4) final filling during atrial systole. The isovolumic relaxation phase is energy dependent. During auxotonic relaxation (phases 2 through 4), ventricular filling occurs against pressure. It encompasses a period during which the myocardium is unable to generate force, and filling of the ventricular chambers takes place. The isovolumic relaxation phase does not contribute to ventricular filling. The greatest amount of ventricular filling occurs in the second phase, whereas the third phase adds only approximately 5% of total diastolic volume and the final phase provides 15% of ventricular volume from atrial systole.
To assess diastolic function, several indices have been developed. The most widely used index for examining the isovolumic relaxation phase of diastole is to calculate the peak instantaneous rate of decline in left ventricular pressure (−d P /d t ) or the time constant of isovolumic decline in left ventricular pressure ( τ ). The aortic closing-mitral opening interval and the isovolumic relaxation time and peak rate of left ventricular wall thinning, as determined by echocardiography, have both been used to estimate diastolic function during auxotonic relaxation. Ventricular compliance can be evaluated by pressure-volume relationships to determine function during the auxotonic phases of diastole.
Many different factors influence diastolic function: magnitude of systolic volume, passive chamber stiffness, elastic recoil of the ventricle, diastolic interaction between the two ventricular chambers, atrial properties, and catecholamines. Whereas systolic dysfunction is a reduced ability of the heart to eject, diastolic dysfunction is a decreased ability of the heart to fill. Abnormal diastolic function is now recognized as the predominant cause of the pathophysiologic condition of congestive heart failure.
Ventricular interactions during systole and diastole are internal mechanisms that provide feedback to modulate stroke volume (SV). Systolic ventricular interaction involves the effect of the interventricular septum on the function of both ventricles. Because the interventricular septum is anatomically linked to both ventricles, it is part of the load against which each ventricle has to work. Therefore, any changes in one ventricle will also be present in the other. In diastolic ventricular interaction, dilatation of either the LV or RV will have an impact on effective filling of the contralateral ventricle and thereby modify function.
Preload and Afterload
Preload is the ventricular load at the end of diastole, before contraction has started. First described by Starling, a linear relationship exists between sarcomere length and myocardial force ( Fig. 14.3 ). In clinical practice, surrogate representatives of left ventricular volume such as pulmonary wedge pressure or central venous pressure are used to estimate preload. More direct measures of ventricular volumes can be made using echocardiography.

Afterload is the systolic load on the LV after contraction has begun. Aortic compliance is an additional determinant of afterload. Aortic compliance is the ability of the aorta to give way to systolic forces from the ventricle. Changes in the aortic wall (dilation or stiffness) can alter aortic compliance and thus afterload. Examples of pathologic conditions that alter afterload are aortic stenosis and chronic hypertension. Both impede ventricular ejection, thereby increasing afterload. Aortic impedance, or aortic pressure divided by aortic flow at that instant, is an accurate means of gauging afterload. However, clinical measurement of aortic impedance is invasive. Echocardiography can noninvasively estimate aortic impedance by determining aortic blood flow at the time of its maximal increase. In clinical practice, the measurement of systolic blood pressure is adequate to approximate afterload, provided that aortic stenosis is not present.
Preload and afterload can be thought of as the wall stress that is present at the end of diastole and during left ventricular ejection, respectively. Wall stress is a useful concept because it includes preload, afterload, and the energy required to generate contraction. Wall stress and heart rate are probably the two most relevant indices that account for changes in myocardial O 2 demand. Laplace’s law states that wall stress ( σ ) is the product of pressure ( P ) and radius ( R ) divided by wall thickness ( h ) :
σ=P×R/2h
The ellipsoid shape of the LV allows the least amount of wall stress such that as the ventricle changes its shape from ellipsoid to spherical, wall stress is increased. By using the ratio of the long axis to the short axis as a measure of the ellipsoid shape, a decrease in this ratio would signify a transition from ellipsoid to spherical.
Thickness of the left ventricular muscle is an important modifier of wall stress. For example, in aortic stenosis, afterload is increased. The ventricle must generate a much higher pressure to overcome the increased load opposing systolic ejection of blood. To generate such high performance, the ventricle increases its wall thickness (left ventricular hypertrophy). By applying Laplace’s law, increased left ventricular wall thickness will decrease wall stress, despite the necessary increase in left ventricular pressure to overcome the aortic stenosis ( Fig. 14.4 ). In a failing heart, the radius of the LV increases, thus increasing wall stress.

Frank-Starling Relationship
The Frank-Starling relationship is an intrinsic property of myocardium by which stretching of the myocardial sarcomere results in enhanced myocardial performance for subsequent contractions (see Fig. 14.3 ). In 1895, Otto Frank first noted that in skeletal muscle, the change in tension was directly related to its length, and as pressure changed in the heart, a corresponding change in volume occurred. In 1914, E.H. Starling, using an isolated heart-lung preparation as a model, observed that “the mechanical energy set free on passage from the resting to the contracted state is a function of the length of the muscle fiber.” If a strip of cardiac muscle is mounted in a muscle chamber under isometric conditions and stimulated at a fixed frequency, then an increase in sarcomere length results in an increase in twitch force. Starling concluded that the increased twitch force was the result of a greater interaction of muscle bundles.
Electron microscopy has demonstrated that sarcomere length (2-2.2 μm) is positively related to the amount of actin and myosin cross-bridging and that there is an optimal sarcomere length at which the interaction is maximal. This concept is based on the assumption that the increase in cross-bridging is equivalent to an increase in muscle performance. Although this theory continues to hold true for skeletal muscle, the force-length relationship in cardiac muscle is more complex. When comparing force-strength relationships between skeletal and cardiac muscle, it is noteworthy that the reduction in force is only 10%, even if cardiac muscle is at 80% sarcomere length. The cellular basis of the Frank-Starling mechanism is still being investigated and is briefly discussed later in this chapter. A common clinical application of Starling’s law is the relationship of left ventricular end-diastolic volume and SV. The Frank-Starling mechanism may remain intact even in a failing heart. However, ventricular remodeling after injury or in heart failure may modify the Frank-Starling relationship.
Contractility
Each Frank-Starling curve specifies a level of contractility, or the inotropic state of the heart, which is defined as the work performed by cardiac muscle at any given end-diastolic fiber. Factors that modify contractility will create a family of Frank-Starling curves with different contractility ( Fig. 14.5 ). Factors that modify contractility are exercise, adrenergic stimulation, changes in pH, temperature, and drugs such as digitalis. The ability of the LV to develop, generate, and sustain the necessary pressure for the ejection of blood is the intrinsic inotropic state of the heart.

In isolated muscle, the maximal velocity of contraction (V max ) is defined as the maximal velocity of ejection at zero load. V max is obtained by plotting the velocity of muscle shortening in isolated papillary muscle at varying degrees of force. Although this relationship can be replicated in isolated myocytes, V max cannot be measured in an intact heart because complete unloading is impossible. To measure the intrinsic contractile activity of an intact heart, several strategies have been attempted with varying success. Pressure-volume loops, albeit requiring catheterization of the left side of the heart, are currently the best way to determine contractility in an intact heart ( Fig. 14.6 ). The pressure-volume loop represents an indirect measure of the Frank-Starling relationship between force (pressure) and muscle length (volume). Clinically, the most commonly used noninvasive index of ventricular contractile function is the ejection fraction, which is assessed by echocardiography, angiography, or radionuclide ventriculography.
Ejectionfraction=(LVEDV–LVESV)/LVEDV

Cardiac Work
The work of the heart can be divided into external and internal work. External work is expended to eject blood under pressure, whereas internal work is expended within the ventricle to change the shape of the heart and to prepare it for ejection. Internal work contributes to inefficiency in the performance of the heart. Wall stress is directly proportional to the internal work of the heart.
External work, or stroke work, is a product of the SV and pressure ( P ) developed during ejection of the SV.
Strokework=SV×Por(LVEDV–LVESV)×P
The external work and internal work of the ventricle both consume O 2 . The clinical significance of internal work is illustrated in the case of a poorly drained LV during cardiopulmonary bypass. Although external work is provided by the roller pump during bypass, myocardial ischemia can still occur because poor drainage of the LV creates tension on the left ventricular wall and increases internal work.
The efficiency of cardiac contraction is estimated by the following formula :
Cardiacefficiency=Externalwork/EnergyequivalentofO2consumption
The corkscrew motion of the heart for the ejection of blood is the most favorable in terms of work efficiency, based on the architecture in a normal LV (with the cardiac muscle bundles arranged so that a circumferentially oriented middle layer is sandwiched by longitudinally oriented outer layers). In heart failure, ventricular dilation reduces cardiac efficiency because it increases wall stress, which in turn increases O 2 consumption.
Heart Rate and Force-Frequency Relationship
In isolated cardiac muscle, an increase in the frequency of stimulation induces an increase in the force of contraction. This relationship is termed the treppe , which means staircase in German, and is the phenomenon of the force-frequency relationship. At between 150 and 180 stimuli per minute, maximal contractile force is reached in an isolated heart muscle at a fixed muscle length. Thus, an increased frequency incrementally increases inotropy, whereas stimulation at a lower frequency decreases contractile force. However, when the stimulation becomes extremely rapid, the force of contraction decreases. In the clinical context, pacing-induced positive inotropic effects may be effective only up to a certain heart rate, based on the force-frequency relationship. In a failing heart, the force-frequency relationship may be less effective in producing a positive inotropic effect.
Cardiac Output
Cardiac output is the amount of blood pumped by the heart per unit of time ( <SPAN role=presentation tabIndex=0 id=MathJax-Element-5-Frame class=MathJax style="POSITION: relative" data-mathml='Q˙’>?˙Q˙
Q ˙
) and is determined by four factors: two factors that are intrinsic to the heart—heart rate and myocardial contractility—and two factors that are extrinsic to the heart but functionally couple the heart and the vasculature—preload and afterload.
Heart rate is defined as the number of beats per minute and is mainly influenced by the autonomic nervous system. Increases in heart rate escalate cardiac output if ventricular filling is adequate during diastole. Contractility can be defined as the intrinsic level of contractile performance that is independent of loading conditions. Contractility is difficult to define in an intact heart because it cannot be separated from loading conditions. For example, the Frank-Starling relationship is defined as the change in intrinsic contractile performance, based on changes in preload. Cardiac output in a living organism can be measured with the Fick principle (a schematic depiction is illustrated in Fig. 14.7 ).

The Fick principle is based on the concept of conservation of mass such that the O 2 delivered from pulmonary venous blood ( q 3 ) is equal to the total O 2 delivered to pulmonary capillaries through the pulmonary artery ( q 1 ) and the alveoli ( q 2 ).
The amount of O 2 delivered to the pulmonary capillaries by way of the pulmonary arteries ( q 1 ) equals total pulmonary arterial blood flow ( <SPAN role=presentation tabIndex=0 id=MathJax-Element-6-Frame class=MathJax style="POSITION: relative" data-mathml='Q˙’>?˙Q˙
Q ˙
) times the O 2 concentration in pulmonary arterial blood (CpaO 2 ):
q1=Q˙×CpaO2
The amount of O 2 carried away from pulmonary venous blood ( q 3 ) is equal to total pulmonary venous blood flow ( <SPAN role=presentation tabIndex=0 id=MathJax-Element-8-Frame class=MathJax style="POSITION: relative" data-mathml='Q˙’>?˙Q˙
Q ˙
) times the O 2 concentration in pulmonary venous blood (Cpv O 2 ):
q3=Q˙×CpvO2
The pulmonary arterial O 2 concentration is the mixed systemic venous O 2 , and the pulmonary venous O 2 concentration is the peripheral arterial O 2 . O 2 consumption is the amount of O 2 delivered to the pulmonary capillaries from the alveoli ( q 2 ). Because q 1 + q 2 = q 3 ,
Q˙(CpaO2)+q2=Q˙(CpvO2)q2=Q˙(CpvO2)−Q˙(CpaO2)q2=Q˙(CpvO2)−(CpaO2)Q˙=q2/(CpvO2−CpaO2)
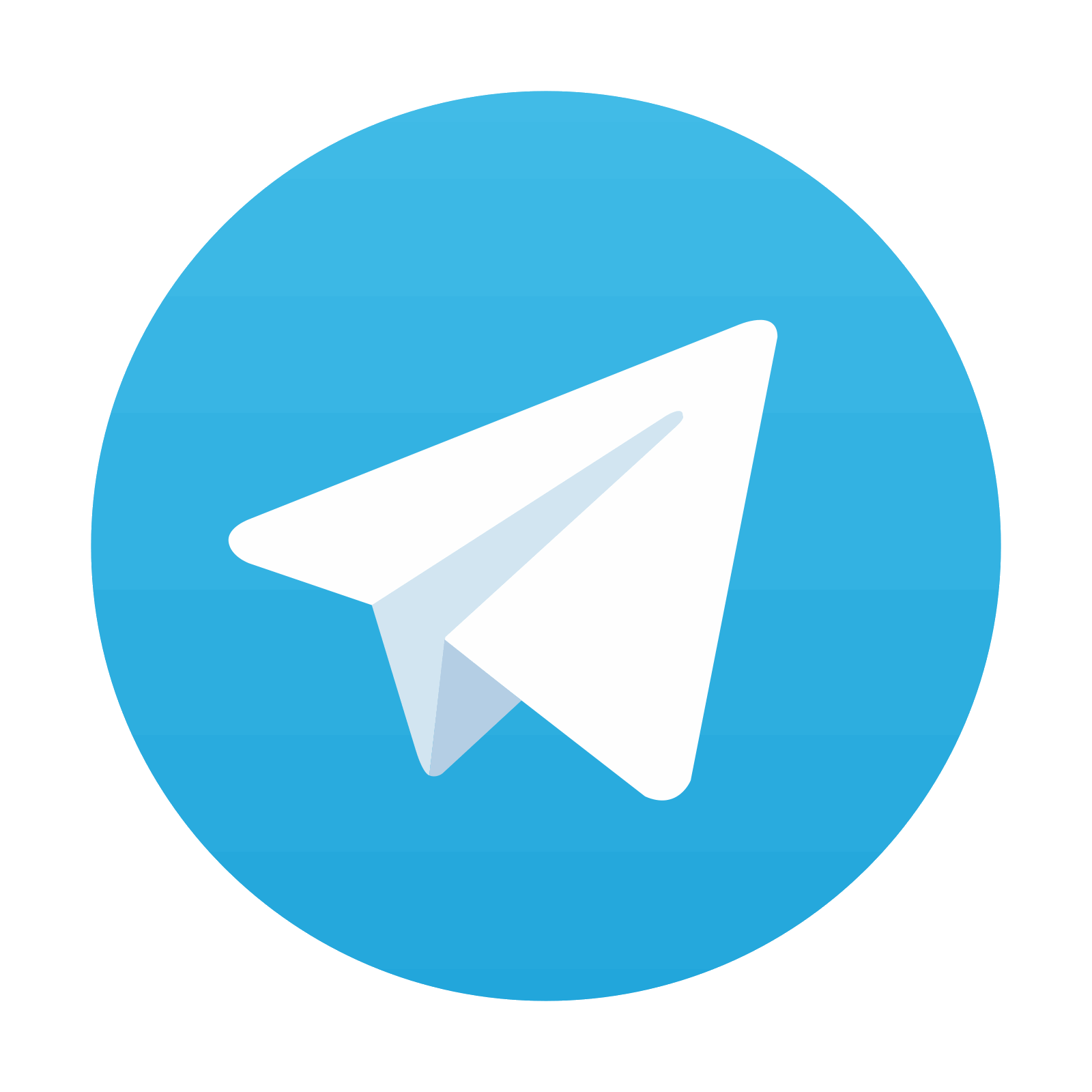
Stay updated, free articles. Join our Telegram channel
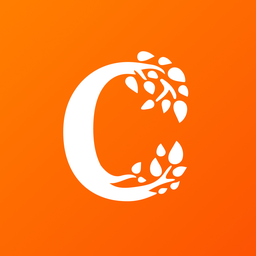
Full access? Get Clinical Tree
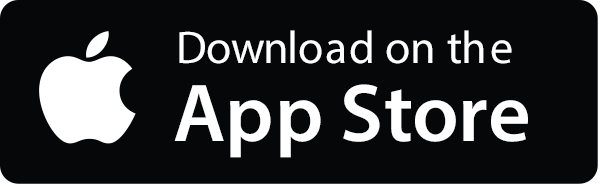
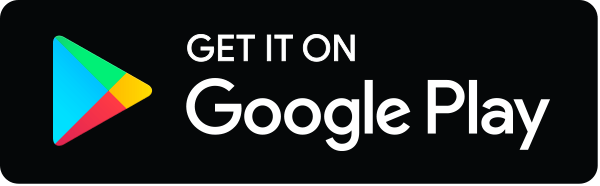
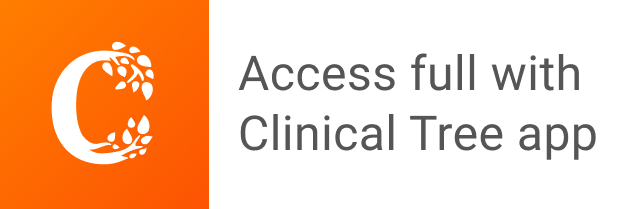