Key points
- •
The spine protects the spinal cord and provides mechanical strength to the trunk while allowing for a certain amount of flexibility.
- •
The intervertebral disc has a design and structure highly optimized to fulfill its mechanical requirements, that is, to sustain the compressive loads arising from the erect posture and distribute them to the vertebrae.
- •
Zygapophyseal joints, also known as facet joints, limit the spinal motion especially in extension and torsion to protect the spinal cord and the nerve roots from pinching and excessive strains.
- •
The mechanical effects of the degenerative changes of the human spine have been widely discussed in the scientific literature, but controversial results have been reported.
Introduction
The spine is the characterizing anatomical structure of the vertebrates and is indeed a shared element in the body design of a high number of extant animals. In humans, the spine had evolved to fulfill the requirements of bipedal stance and gait [ ], within the evolutionary constraints of the tetrapod body plan and a somehow fixed, genetically determined structure composed by an anterior vertebral body, pedicles and laminae defining a canal to host the spinal cord, and various processes that provide insertion points for ligaments and muscles [ , ].
In the following paragraphs, the biomechanics of the human spine is illustrated by describing the functional anatomy and mechanical role of its various components, namely vertebrae, intervertebral discs (IVDs), facet joints, and ligaments, highlighting the anatomical and biomechanical differences between humans and other vertebrates whenever relevant. Finally, the biomechanical impact of spinal phenotypes associated with the degenerative process in aging subjects is analyzed.
The spinal anatomy in vertebrates and humans
The earliest chordates, ancestors of vertebrates that possessed a notochord and in some cases rudimentary vertebrae, appeared more than 500 million years ago [ , ], and later evolved into fish and amphibians, which developed four functional limbs for terrestrial locomotion. The tetrapod body plan emerged as the most successful strategy for nonaquatic life from an evolutionary point of view, and it still characterizes amphibians, reptiles, birds, and mammals [ ].
In most extant vertebrates, the notochord has evolved in a series of bones (vertebrae) connected by soft tissues, called spine or vertebral column, which protects the spinal cord and provides mechanical strength to the trunk while allowing for a certain amount of flexibility to accommodate body motion in all directions [ ]. In mammals, the spine can be generally divided into five regions: in the craniocaudal direction, the cervical, thoracic, lumbar, sacral, and caudal spines. The cervical spine is composed of seven vertebrae (except in sloths and manatees [ ]) and supports the head while permitting its motion. The thoracic spine is characterized by the presence of ribs, and sometimes prominent dorsal and lateral appendages named spinous and transverse processes. Lumbar vertebrae connect the trunk to the pelvis, and their number is rather variable among species. Whereas whales have up to 20 bony segments [ ], most apes have only four lumbar vertebrae. The number of lumbar vertebrae increased in hominins (australopithecines had 5 or 6) [ ], and is typically 5 in modern humans. Some mammals that are believed to be good models to study the biomechanics of the human spine, such as sheep, calves, and pigs have six or seven lumbar vertebrae [ ]. Sacral vertebrae are fused in many mammals (such as primates) in a single sacral bone connected to the pelvic girdle [ ]. Caudal vertebrae are also variable in number and rather simpler in shape; they usually do not have a neural canal as the spinal cord and nerve roots usually end in the lumbosacral spine. In humans and other primates, caudal vertebrae are fused together in the so-called coccyx.
The intervertebral disc
In mammals and birds, the upper and lower surfaces of adjacent vertebral bodies, named vertebral endplates, are mechanically connected by means of the IVD, a fibrocartilaginous structure that distributes the spinal loads and limits the flexibility of each motion segment. The IVD has a design and structure highly optimized to fulfill its mechanical requirements; in humans, such requirements consist in sustaining the compressive loads arising from the erect posture and distributing them to the surrounding bony structures through the vertebral endplates [ , ] ( Fig. 2.1 ). The inner nucleus pulposus (NP) has high water content (80%–90%) and is able to sustain high pressures [ ]. Due to its fluid-like nature, its inner stresses do not have a preferential orientation and are therefore hydrostatic [ ]. Similarly to a tyre, the NP is contained by an external fiber-reinforced shell, the annulus fibrosus (AF), which is loaded in tension when the disc is subjected to compression and limits the bulging of the inner core [ ] ( Fig. 2.2A ). Due to its high proteoglycan content, the IVD has the capability to swell [ ], that is, to attract fluid from the external environment and therefore to possess an intrinsic internal pressure even in the absence of load, which prevents collapse and inward bulging of the annular fibers [ ].


If in healthy conditions, the optimized structure of the IVD was shown to be capable to provide a nearly homogeneous stress state along the vertebral endplates under physiological loads [ ] avoiding stress peaks that may support degenerative changes leading to endplate defects. Indeed, disc degeneration, which determines high-stress concentrations on the endplates [ , ], was proven to be the most important predictor of the presence of Schmorl’s nodes [ ], that is, a nuclear protrusion inside the adjacent vertebral bodies.
The geometry of the vertebral endplates has been shown to contribute to the even stress distribution and therefore to limit the risk of endplate failures [ ]. A recent study comparing the shape of vertebral endplates in healthy humans, humans with endplate defects, and extant apes showed that human vertebrae exhibiting a Schmorl’s node had endplates with shapes more similar to those of chimpanzees and orangutans, which are arguably less adapted to bipedalism [ ]. Indeed, human vertebrae with Schmorl’s nodes had rounder, shovel-shaped IVD profiles similar to those of chimpanzees and possibly more adapted to tensional loads acting during arm swinging, whereas healthy humans had a heart-shape profile that was hypothesized to be more efficient in sustaining compressive loads. Furthermore, pathological vertebrae showed smaller neural foramina as well as shorted and wider pedicles, all features that are found also in apes.
The powerful human back musculature and the long lever arms of the spine muscles, in combination with the action of the trunk weight, determine a high loading and stress condition in the IVDs [ ]. In both apes and humans, loads acting in the IVD are in the axial direction, but whereas arm swinging may involve significant tensile stresses, bipedal stance and gait mostly induce compressive loads in the intervertebral discs [ ]. In vivo measurements with pressure transducers implanted in the lumbar spine of human volunteers showed that the IVD is indeed subjected to high intradiscal pressures, which are strongly variable in different postures and activities such as walking and weight lifting [ , ] ( Table 2.1 ).
Position | Pressure (MPa) | % |
---|---|---|
Lying supine | 0.1 | 20 |
Side-lying | 0.12 | 24 |
Lying prone | 0.11 | 22 |
Peaks by turning around during bed rest | 0.7–0.8 | 140–160 |
Relaxed standing | 0.5 | 100 |
Standing, bent forward | 1.1 | 220 |
Sitting relaxed, without backrest | 0.46 | 92 |
Sitting in maximum flexion | 0.83 | 166 |
Sitting slouched into the chair | 0.27 | 54 |
Standing up from the chair | 1.1 | 220 |
Walking barefoot | 0.53–0.65 | 106–130 |
Jogging | 0.35–0.95 | 70–190 |
Climbing stairs | 0.5–0.7 | 100–140 |
Walking downstairs | 0.38–0.6 | 76–120 |
Lifting 20 kg, bent over with round back | 2.3 | 460 |
Lifting 20 kg as taught in back school | 1.7 | 340 |
Holding 20 kg close to the body | 1.1 | 220 |
Holding 20 kg, 60 cm away from the chest | 1.8 | 360 |
Carrying 20 kg (in the left or in the right hand) | 1.0 | 200 |
Carrying 40 kg (20 kg left and 20 kg right) | 0.9 | 180 |
Pressure increase during bed rest (over a period of 7 h) | 0.1–0.24 | 20–48 |
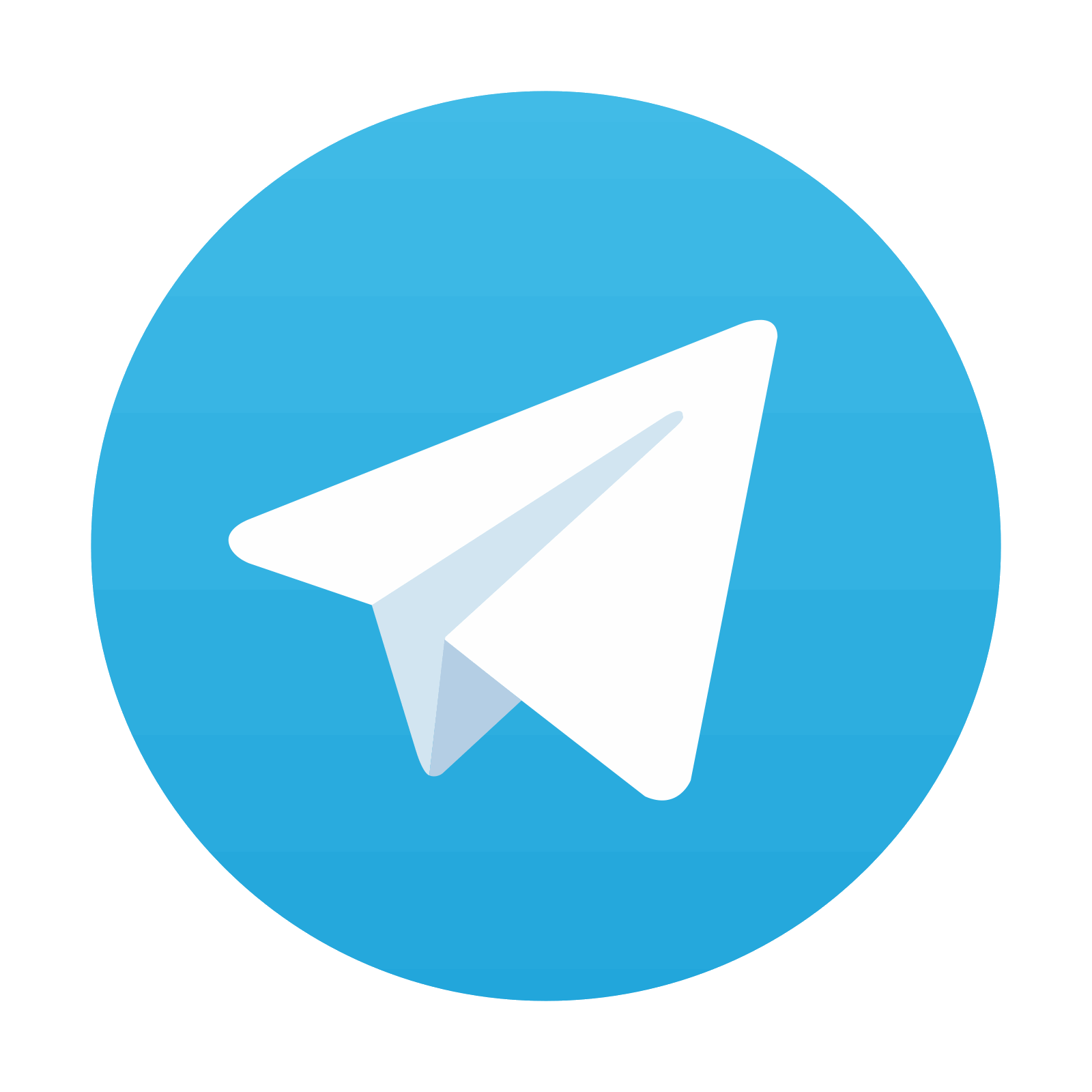
Stay updated, free articles. Join our Telegram channel
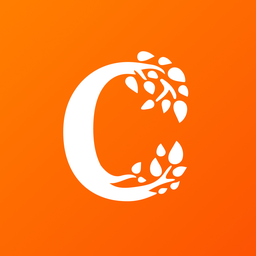
Full access? Get Clinical Tree
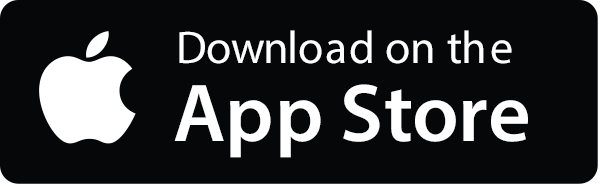
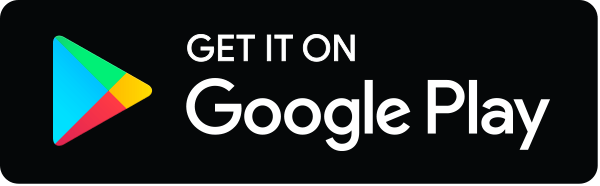
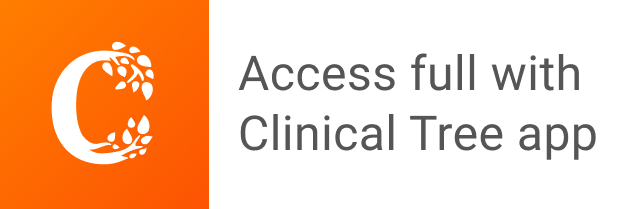