This simple view helps to understand the action of an antagonist (Fig. 2-2). An antagonist is a drug that binds to the receptor without activating the receptor. Antagonists typically bind with ionic, hydrogen, and van der Waals interactions, rendering them reversible. Antagonists block the action of agonists simply by getting in the way of the agonist, preventing the agonist from binding to the receptor and producing the drug effect. Competitive antagonism is present when increasing concentrations of the antagonist progressively inhibit the response to the agonist. This causes a rightward displacement of the agonist dose-response (or concentration-response) relationship. Noncompetitive antagonism is present when, after administration of an antagonist, even high concentrations of agonist cannot completely overcome the antagonism. In this instance, either the agonist is bound irreversibly (and probably covalently) to the receptor site, or it binds to a different site on the molecule and the interaction is allosteric (based on a change in shape and thereby the activity of the receptor). Noncompetitive antagonism causes both a rightward shift of the dose-response relationship as well as a decreased maximum efficacy of the concentration versus response.

Although this simple view of activated and inactivated receptors explains agonists and antagonists, it has a more difficult time with partial agonists and inverse agonists (Fig. 2-3). A partial agonist is a drug that binds to a receptor (usually at the agonist site) where it activates the receptor but not as much as a full agonist. Even at supramaximal doses, a partial agonist cannot cause the full drug effect. Partial agonists may also have antagonist activity in which case they are also called agonist-antagonists. When a partial agonist is administered with a full agonist, it decreases the effect of the full agonist. For example, butorphanol acts as a partial agonist at the µ opioid receptor. Given alone, butorphanol is a modestly efficacious analgesic. Given along with fentanyl, it will partly reverse the fentanyl analgesia, and in individuals using opioids chronically, may precipitate withdrawal. Inverse agonists bind at the same site as the agonist (and likely compete with it), but they produce the opposite effect of the agonist. Inverse agonists “turn off” the constitutive activity of the receptor. The simple view of receptors as bound or unbound does not explain partial agonists or inverse agonists.

It turns out that receptors have many natural conformations, and they naturally fluctuate between these different conformations (Fig. 2-4). Some of the conformations are associated with the pharmacologic effect, and some are not. In the example shown, the receptor only has two states: an inactive state and an active state that produces the same effect as if an agonist were bound to the receptor, although at a reduced level because the receptor only spends 20% of its time in this activated state.

In this view, ligands do not cause the receptor shape to change. That happens spontaneously. However, ligands change the ratio of active to inactive states by (thermodynamically) favoring one of the states. Figure 2-5 shows the receptor as seen in Figure 2-4 in the presence of an agonist, a partial agonist, an antagonist, and an inverse agonist. Presence of the full agonist causes the conformation of the active state to be strongly favored, causing the receptors to be in this state nearly 100% of the time. The partial agonist is not as effective in stabilizing the receptor in the active state, so the bound receptor only spends 50% of its time in this state. The antagonist does not favor either state; it just gets in the way of binding (as before; see Fig. 2-2). The inverse agonist favors the inactive state, reversing the baseline receptor activity.

Using this information, we can now interpret the action of several ligands for the benzodiazepine receptor (see Fig. 2-3). The actions include full agonism (midazolam), partial agonism (bretazenil), competitive antagonism (flumazenil), and inverse agonism (RO 19-4063). This range of actions can be explained by considering receptor states. Assume that the γ-aminobutyric acid (GABA) receptor has several conformations, one of which is particularly sensitive to endogenous GABA. Typically, there are some GABA receptors in this more sensitive conformation. As a full agonist, midazolam causes nearly all of the GABA receptors to be in the confirmation with increased sensitivity to GABA. Bretazenil does the same thing but not as well. Even when every benzodiazepine receptor is occupied by bretazenil, fewer GABA receptors are in the more sensitive confirmation. Bretazenil simply does not favor that conformation as well as midazolam. When flumazenil is in the binding pocket, it does not change the relative probabilities of the receptor being in any conformation. Flumazenil just gets in the way of other drugs that would otherwise bind to the pocket. RO 19-4063 actually decreases the number of GABA receptors in the more sensitive conformation. Usually, some of them are in this more sensitive conformation, but that number is decreased by the inverse agonist RO 19-4063 (which was never developed as a drug because endogenous benzodiazepines, although anticipated, have not been described). The notion of the drugs having multiple conformations, and drugs acting through favoring particular conformations, helps to understand the action of agonists, partial agonists, antagonists, and inverse agonists.
Receptor Action
The number for receptors in cell membranes is dynamic and increases (upregulates) or decreases (downregulates) in response to specific stimuli. For example, a patient with pheochromocytoma has an excess of circulating catecholamines. In response, there is a decrease in the numbers of β-adrenergic receptors in cell membranes in an attempt to maintain homeostasis. Likewise, prolonged treatment of asthma with a β agonist may result in tachyphylaxis (decreased response to the same dose of β agonist, also called tolerance) because of the decrease in β adrenergic receptors. Conversely, lower motor neuron injury causes an increase in the number of nicotinic acetylcholine receptors in the neuromuscular junction, leading to an exaggerated response to succinylcholine. Changing receptor numbers is one of many mechanisms that contribute to variability in response to drugs.
Receptor Types
Receptors for drug action can be classified by location. Many of the receptors thought to be the most critical for anesthetic interaction are located in the lipid bilayer of cell membranes. For example, opioids, intravenous sedative hypnotics, benzodiazepines, β blockers, catecholamines, and muscle relaxants (most of which are actually antagonists) all interact with membrane-bound receptors. Other receptors are intracellular proteins. Drugs such as caffeine, insulin, steroids, theophylline, and milrinone interact with intracellular proteins. Circulating proteins can also be drug targets; for example, the many drugs that affect components of the coagulation cascade.
There are also drugs that do not interact with proteins at all. Stomach antacids such as sodium citrate simply work by changing gastric pH. Chelating drugs work by binding divalent cations. Iodine kills bacteria by osmotic pressure (intracellular desiccation), and intravenous sodium bicarbonate changes plasma pH. The mechanism of action of these drugs does not involve receptors per se, and hence these drugs will not be further considered in this section.
Proteins function in the body as small machines, catalyzing enzymatic reactions and acting as ion channels among other functions. When a drug binds to a receptor, it changes the activity of the machine, typically by enhancing its activity (e.g., propofol increases the sensitivity of the GABA-A receptor to GABA, the endogenous ligand), decreasing its activity (ketamine decreases the activity of the N-methyl-D-aspartate [NMDA] receptor), or triggering a chain reaction (opioid binding to the µ opioid receptor activates an inhibitory G protein that decreases adenylyl cyclase activity). The protein’s response to binding of the drug is responsible for the drug effect.
Pharmacokinetics
Pharmacokinetics is the quantitative study of the absorption, distribution, metabolism, and excretion of injected and inhaled drugs and their metabolites. Thus, pharmacokinetics describes what the body does to a drug. Pharmacodynamics is the quantitative study of the body’s response to a drug. Thus, pharmacodynamics describes what the drug does to the body. This section will introduce the basic principles of pharmacokinetics. The next section discusses the basic principles of pharmacodynamics.
Pharmacokinetics determines the concentration of a drug in the plasma or at the site of drug effect. Pharmacokinetic variability is a significant component of patient-to-patient variability in drug response and may result from genetic modifications in metabolism; interactions with other drugs; or disease in the liver, kidneys, or other organs of metabolism.4
The basic principles of pharmacokinetics are absorption, metabolism, distribution, and elimination. These processes are fundamental to all drugs. They can be described in basic physiologic terms or using mathematical models. Each serves a purpose. Physiology can be used to predict how changes in organ function will affect the disposition of drugs. Mathematical models can be used to calculate the concentration of drug in the blood or tissue following any arbitrary dose, at any arbitrary time. We will initially tackle the physiologic principles that govern distribution, metabolism, elimination, and absorption, in that order. We will then turn to the mathematical models.
Distribution
When drugs are administered, they mix with body tissues and are immediately diluted from the concentrated injectate in the syringe to the more dilute concentration measured in the plasma or tissue. This initial distribution (within 1 minute) after bolus injection is considered mixing within the “central compartment” (Fig. 2-6). The central compartment is physically composed of those elements of the body that dilute the drug within the first minute after injection: the venous blood volume of the arm, the volume of the great vessels, the heart, the lung, and the upper aorta, and whatever uptake of drug occurs in the first passage through the lungs. Many of these volumes are fixed, but drugs that are highly fat soluble may be avidly taken up in the first passage through the lung, reducing the concentration measured in the arterial blood and increasing the apparent size of the central compartment. For example, first-pass pulmonary uptake of the initial dose of lidocaine, propranolol, meperidine, fentanyl, sufentanil, and alfentanil exceeds 65% of the dose.5

The body is a complex space, and mixing is an ongoing process. Almost by definition, the central compartment is the mixing with a small portion of the blood volume and the lung tissue. Several minutes later, the drug will fully mix with the entire blood volume. However, it may take hours or even days for the drug to fully mix with all bodily tissues because some tissues have very low perfusion.
In the process of mixing, molecules are drawn to other molecules, some with specific binding sites. A drug that is polar will be drawn to water, where the polar water molecules find a low energy state by associating with the charged aspects of the molecule. A drug that is nonpolar has a higher affinity for fat, where van der Waals binding provides numerous weak binding sites. Many anesthetic drugs are highly fat soluble and poorly soluble in water. High fat solubility means that the molecule will have a large volume of distribution because it will be preferentially taken up by fat, diluting the concentration in the plasma. The extreme example of this is propofol, which is almost inseparable from fat. The capacity of body fat to hold propofol is so vast that in some studies the total volume of distribution of propofol has been reported as exceeding 5,000 L. Of course, nobody has a total volume of 5,000 L. It is important to understand that those 5,000 L refer to imaginary aqueous liters or the amount of plasma that would be required to dissolve the initial dose of propofol. Because propofol is so fat soluble, a large amount of propofol is dissolved in the body’s fatty tissues and the concentration measured in the plasma will be low.
Following bolus injection, the drug primarily goes to the tissues that receive the bulk of arterial blood flow: the brain, heart, kidneys, and liver. These tissues are often called the vessel rich group. The rapid blood flow ensures that the concentration in these highly perfused tissues rises rapidly to equilibrate with arterial blood. However, for highly fat soluble drugs, the capacity of the fat to hold the drug greatly exceeds the capacity of highly perfused tissues. Initially, the fat compartment is almost invisible because the blood supply to fat is quite limited. However, with time, the fat gradually absorbs more and more drug, sequestering it away from the highly perfused tissues. This redistribution of drug from the highly perfused tissue to the fat accounts for a substantial part of the offset of drug effect following a bolus of an intravenous anesthetic or fat-soluble opioid (e.g., fentanyl). Muscles play an intermediate role in this process, having (at rest) blood flow that is intermediate between highly perfused tissues and fat.
Protein Binding
Most drugs are bound to some extent to plasma proteins, primarily albumin, α1-acid glycoprotein, and lipoproteins.6 Most acidic drugs bind to albumin, whereas basic drugs bind to α1-acid glycoprotein. Protein binding effects both the distribution of drugs (because only the free or unbound fraction can readily cross cell membranes) and the apparent potency of drugs, again because it is the free fraction that determines the concentration of bound drug on the receptor.
The extent of protein binding parallels the lipid solubility of the drug. This is because drugs that are hydrophobic are more likely to bind to proteins in the plasma and to lipids in the fat. For intravenous anesthetic drugs, which tend to be quite potent, the number of available protein binding sites in the plasma vastly exceeds the number of sites actually bound. As a result, the fraction bound is not dependent on the concentration of the anesthetic and only dependent on the protein concentration.
Binding of drugs to plasma albumin is nonselective, and drugs with similar physicochemical characteristics may compete with each other and with endogenous substances for the same protein binding sites. For example, sulfonamides can displace unconjugated bilirubin from binding sites on albumin, leading to the risk of bilirubin encephalopathy in the neonate.
Age, hepatic disease, renal failure, and pregnancy can all result in decreased plasma protein concentration. Alterations in protein binding are important only for drugs that are highly protein bound (e.g., >90%). For such drugs, the free fraction changes as an inverse proportion with a change in protein concentration. If the free fraction is 2% in the normal state, then in a patient with 50% decrease in plasma proteins, the free fraction will increase to 4%, a 100% increase.
Theoretically, an increase in free fraction of a drug may increase the pharmacologic effect of the drug, but in practice, it is far from certain that there will be any change in pharmacologic effect at all. The reason is that it is the unbound fraction that equilibrates throughout the body, including with the receptor. Plasma proteins only account for a small portion of the total binding sites for drug in the body. Because the free drug concentration in the plasma and tissues represents partitioning with all binding sites, not just the plasma binding sites, the actual free drug concentration that drives drug on and off receptors may change fairly little with changes in plasma protein concentration.
Metabolism
Metabolism converts pharmacologically active, lipid-soluble drugs into water-soluble and usually pharmacologically inactive metabolites. However, this is not always the case. For example, diazepam and propranolol may be metabolized to active compounds. Morphine-6-glucuronide, a metabolite of morphine, is a more potent opioid than morphine itself. In some instances, an inactive parent compound (prodrug) metabolized to an active drug. This is the case with codeine, which is an exceedingly weak opioid. Codeine is metabolized to morphine, which is responsible for the analgesic effects of codeine.
Pathways of Metabolism
The four basic pathways of metabolism are (a) oxidation, (b) reduction, (c) hydrolysis, and (d) conjugation. Traditionally, metabolism has been divided into phase I and phase II reactions. Phase I reactions include oxidation, reduction, and hydrolysis, which increase the drug’s polarity and prepare it for phase II reactions. Phase II reactions are conjugation reactions that covalently link the drug or metabolites with a highly polar molecule (carbohydrate or an amino acid) that renders the conjugate more water soluble for subsequent excretion. Hepatic microsomal enzymes are responsible for the metabolism of most drugs. Other sites of drug metabolism include the plasma (Hofmann elimination, ester hydrolysis), lungs, kidneys, and gastrointestinal tract and placenta (tissue esterases).
Hepatic microsomal enzymes, which participate in the metabolism of many drugs, are located principally in hepatic smooth endoplasmic reticulum. These microsomal enzymes are also present in the kidneys, gastrointestinal tract, and adrenal cortex. Microsomes are vesicle-like artifacts re-formed from pieces of the endoplasmic reticulum when cells are homogenized; microsomal enzymes are those enzymes that are concentrated in these vesicle-like artifacts.
Phase I Enzymes
Enzymes responsible for phase I reactions include cytochrome P450 enzymes, non–cytochrome P450 enzymes, and flavin-containing monooxygenase enzymes. The cytochrome P450 enzyme (CYP) system is a large family of membrane-bound proteins containing a heme cofactor that catalyze the metabolism of endogenous compounds. P450 enzymes are predominantly hepatic microsomal enzymes although there are also mitochondrial P450 enzymes. The designation cytochrome P450 emphasizes this substance’s absorption peak at 450 nm when it is combined with carbon monoxide. The cytochrome P450 system is also known as the mixed function oxidase system because it involves both oxidation and reduction steps; the most common reaction catalyzed by cytochrome P450 is the monooxygenase reaction, for example, insertion of one atom of oxygen into an organic substrate while the other oxygen atom is reduced to water. Cytochrome P450 functions as the terminal oxidase in the electron transport chain.
Individual cytochrome P450 enzymes have evolved from a common protein.7 Cytochrome P450 enzymes, often called CYPs, that share more than 40% sequence homology are grouped in a family designated by a number (e.g., “CYP2”), those that share more than 55% homology are grouped in a subfamily designated by a letter (e.g., “CYP2A”), and individual CYP enzymes are identified by a third number (e.g., “CYP2A6”). Ten isoforms of cytochrome P450 are responsible for the oxidative metabolism of most drugs. The preponderance of CYP activity for anesthetic drugs is generated by CYP3A4, which is the most abundantly expressed P450 isoform, comprising 20% to 60% of total P450 activity. P450 3A4 metabolizes more than one-half of all currently available drugs, including opioids (alfentanil, sufentanil, fentanyl), benzodiazepines, local anesthetics (lidocaine, ropivacaine), immunosuppressants (cyclosporine), and antihistamines (terfenadine).
Drugs can alter the activity of these enzymes through induction and inhibition. Induction occurs through increased expression of the enzymes. For example, phenobarbital induces microsomal enzymes and thus can render drugs less effective through increased metabolism. Conversely, other drugs directly inhibit enzymes, increasing the exposure to their substrates. Famously, grapefruit juice (not exactly a drug) inhibits CYP 3A4, possibly increasing the concentration of anesthetics and other drugs.
Oxidation
Cytochrome P450 enzymes are crucial for oxidation reactions. These enzymes require an electron donor in the form of reduced nicotinamide adenine dinucleotide (NAD) and molecular oxygen for their activity. The molecule of oxygen is split, with one atom of oxygen oxidizing each molecule of drug and the other oxygen atom being incorporated into a molecule of water. Examples of oxidative metabolism of drugs catalyzed by cytochrome P450 enzymes include hydroxylation, deamination, desulfuration, dealkylation, and dehalogenation. Demethylation of morphine to normorphine is an example of oxidative dealkylation. Dehalogenation involves oxidation of a carbon-hydrogen bond to form an intermediate metabolite that is unstable and spontaneously loses a halogen atom. Halogenated volatile anesthetics are susceptible to dehalogenation, leading to release of bromide, chloride, and fluoride ions. Aliphatic oxidation is oxidation of a side chain. For example, oxidation of the side chain of thiopental converts the highly lipid-soluble parent drug to the more water-soluble carboxylic acid derivative. Thiopental also undergoes desulfuration to pentobarbital by an oxidative step.
Epoxide intermediates in the oxidative metabolism of drugs are capable of covalent binding with macromolecules and may be responsible for some drug-induced organ toxicity, such as hepatic dysfunction. Normally, these highly reactive intermediates have such a transient existence that they exert no biologic action. When enzyme induction occurs, however, large amounts of reactive intermediates may be produced, leading to organ damage. This is especially likely to occur if the antioxidant glutathione, which is in limited supply in the liver, is depleted by the reactive intermediates.
Reduction
Cytochrome P450 enzymes are also essential for reduction reactions. Under conditions of low oxygen partial pressures, cytochrome P450 enzymes transfer electrons directly to a substrate such as halothane rather than to oxygen. This electron gain imparted to the substrate occurs only when insufficient amounts of oxygen are present to compete for electrons.
Conjugation
Conjugation with glucuronic acid involves cytochrome P450 enzymes. Glucuronic acid is synthesized from glucose and added to lipid-soluble drugs to render them water soluble. The resulting water-soluble glucuronide conjugates are then excreted in bile and urine. In premature infants, reduced microsomal enzyme activity interferes with conjugation, leading to neonatal hyperbilirubinemia and the risk of bilirubin encephalopathy. The reduced conjugation ability of the neonate increases the effect and potential toxicity of drugs that are normally inactivated by conjugation with glucuronic acid.
Hydrolysis
Enzymes responsible for hydrolysis of drugs, usually at an ester bond, do not involve the cytochrome P450 enzyme system. Hydrolysis often occurs outside of the liver. For example, remifentanil, succinylcholine, esmolol, and the ester local anesthetics are cleared in the plasma and tissues via ester hydrolysis.
Phase II Enzymes
Phase II enzymes include glucuronosyltrasferases, glutathione-S-transferases, N-acetyl-transferases, and sulfotransferases. Uridine diphosphate glucuronosyltransferase catalyzes the covalent addition of glucuronic acid to a variety of endogenous and exogenous compounds, rendering them more water soluble. Glucuronidation is an important metabolic pathway for several drugs used during anesthesia, including propofol, morphine (yielding morphine-3-glucuronide and the pharmacologically active morphine-6-glucuronide), and midazolam (yielding the pharmacologically active 1-hydroxymidazolam). Glutathione-S-transferase (GST) enzymes are primarily a defensive system for detoxification and protection against oxidative stress. N-acetylation catalyzed by N-acetyl-transferase (NAT) is a common phase II reaction for metabolism of heterocyclic aromatic amines (particularly serotonin) and arylamines, including the inactivation of isoniazid.
Hepatic Clearance
The rate of metabolism for most anesthetic drugs is proportional to drug concentration, rending the clearance of the drug constant (i.e., independent of dose). This is a fundamental assumption for anesthetic pharmacokinetics. Exploring this assumption will provide insight into what clearance actually is and how it relates to the metabolism of drugs.
Although the metabolic capacity of the body is large, it is not possible that metabolism is always proportional to drug concentration because the liver does not have infinite metabolic capacity. At some rate of drug flow into the liver, the organ will be metabolizing drug as fast as the metabolic enzymes in the organ allow. At this point, metabolism can no longer be proportional to concentration because the metabolic capacity of the organ has been exceeded.
Understanding metabolism starts with a simple mass balance: the rate at which drug flows out of the liver must be the rate at which drug flows into the liver, minus the rate at which the liver metabolizes drug. The rate at which drug flows into the liver is liver blood flow, Q, times the concentration of drug flowing in, Cinflow. The rate at which drug flows out of the liver is liver blood flow, Q, times the concentration of drug flowing out, Coutflow. The rate of hepatic metabolism by the liver, R, is the difference between the drug concentration flowing into the liver and the drug concentration flowing out of the liver, times the rate of liver blood flow:

This relationship is illustrated in Figure 2-7.

Metabolism can be saturated because the liver does not have infinite metabolic capacity. A common equation used for this saturation processes is:

“Response” in Equation 2-2 varies from 0 to 1, depending on the value of C. In this context, Response is the fraction of maximal metabolic rate. Response = 0 means no metabolism, and Response = 1 means metabolism at the maximal possible rate. C refers to whatever is driving the response. In this chapter, C means drug concentration. When C is 0, the response is 0. If C is greater than 0 but much less than C50, the denominator is approximately C50 and the response is nearly proportional to C: . If we increase C even further to exactly C50, then the response is
, which is simply 0.5. That is where the name “C50” comes from: it is the concentration associated with 50% response. As C becomes much greater than C50, the equation approaches
, which is 1. The shape of this relationship is shown in Figure 2-8. The relationship is nearly linear at low concentrations, but at high concentrations, the response saturates at 1.

To understand hepatic clearance, we must understand the relationship between hepatic metabolism and drug concentration. But what concentration determines the rate of metabolism: the concentration flowing into the liver, the average concentration within the liver, or the concentration flowing out of the liver? All have been used, but the most common views the rate of metabolism as a function of the concentration flowing out of the liver, Coutflow.
We can expand our equation of metabolism to include the observation that the rate of metabolism, R, approaches saturation at the maximum metabolic rate, Vm, as a function of Coutflow:

The saturation equation appears at the end of the aforementioned equation. Vm is the maximum possible metabolic rate. The saturation part of this equation, , determines fraction of the maximum metabolic rate. Km, the “Michaelis constant,” is the outflow concentration at which the metabolic rate is 50% of the maximum rate (Vm). This relationship is shown in Figure 2-9. The x-axis is the outflow concentration, Coutflow, as a fraction Km. The y-axis is the rate of drug metabolism as a fraction of Vm. By normalizing the x– and y-axis in this manner, the relationship shown in Figure 2-9 is true for all values of Vm and Km. As long as the outflow concentration is less than one-half of Km (true for almost all anesthetic drugs), there is a nearly proportional change in metabolic rate with a proportional change in outflow concentration. Another interpretation is that metabolism will be proportional to concentration as long as the metabolic rate is less than one-third of the maximum metabolic capacity.

So far, we have talked about the rate of metabolism and not about hepatic clearance. If the liver could completely extract the drug from the afferent flow, then clearance would equal liver blood flow, Q. However, the liver cannot remove every last drug molecule. There is always some drug in the effluent plasma. The fraction of inflowing drug extracted by the liver is . This is called the extraction ratio. Clearance is the amount of blood completely cleared of drug per unit time. We can calculate clearance as the liver blood flow times the extraction ratio

With this basic understanding of clearance, let us divide each part of Equation 2-3 by Cinflow:

The third term is clearance as defined in Equation 2-4: Q times the extraction ratio. Thus, each term in Equation 2-4 must be clearance. Let us consider them in order.
The first term tells us that Clearance = . This indicates that clearance is a proportionality constant that relates inflowing (e.g., arterial) concentration to the rate of metabolism. If we want to maintain a given steady-state arterial drug concentration, we must infuse drug at the same rate that it is being metabolized. With this understanding, we can rearrange the equation to say: Infusion rate = metabolic rate = Clearance × Cinflow. Thus, the infusion rate to maintain a given concentration is the clearance times the desired concentration.
The third and fourth terms,

and

are more interesting if taken together. Remembering that is the extraction ratio, these equations relate clearance to liver blood flow and the extraction ratio, as shown in Figure 2-10.8 For drugs with an extraction ratio of nearly 1 (e.g., propofol), a change in liver blood flow produces a nearly proportional change in clearance. For drugs with a low extraction ratio (e.g., alfentanil), clearance is nearly independent of the rate of liver blood flow. This makes intuitive sense. If nearly 100% of the drug is extracted by the liver, then the liver has tremendous metabolic capacity for the drug. In this case, flow of drug to the liver is what limits the metabolic rate. Metabolism is “flow limited.” The reduction in liver blood flow that accompanies anesthesia can be expected to reduce clearance. However, moderate changes in hepatic metabolic function per se will have little impact on clearance because hepatic metabolic capacity is overwhelmingly in excess of demand.

Conversely, for drugs with an extraction ratio considerably less than 1, clearance is limited by the capacity of the liver to take up and metabolize the drug. Metabolism is “capacity limited.” Clearance will change in response to any change in the capacity of the liver to metabolize such drugs, such as might be caused by liver disease or enzymatic induction. However, changes in liver blood flow, as might be caused by the anesthetic state itself, usually have little influence on the clearance because the liver only handles a fraction of the drug it sees. This relationship can be seen in Figure 2-11.

We can also put the third and fourth terms of the clearance equation together to show how extraction ratio governs the response of clearance to changes in metabolic capacity (Vm). Figure 2-11 shows the clearance for drugs with an extraction ratio ranging from 0.1 to 1, based on a liver blood flow of 1.4 L per minute. The extraction ratios were calculated for a Vm = 1. Changes in Vm, as might be caused by liver disease (reduced Vm) or enzymatic induction (increased Vm) have little effect on drugs with a high extraction ratio. However, drugs with a low extraction ratio have a nearly linear change in clearance with a change in intrinsic metabolic capacity (Vm).
Vm and Km are usually not known and condensed into a single term, . This term summarizes the hepatic metabolic capacity and is called intrinsic clearance. Because clearance =
, consider what happens if hepatic blood flow increases to infinity (this is a thought experiment). At super high hepatic blood flow, Coutflow becomes indistinguishable from Cinflow because the finite hepatic capacity only metabolizes an infinitesimal fraction of the drug flowing through the liver. As a result, clearance becomes
. We can solve for this in the “linear range” by finding clearance when Cinflow = 0,
. This is the intrinsic clearance, Clint. It can be demonstrated algebraically from the definition of Clint that in the linear range, Clint is directly related to the extraction ratio:
. Combining this with Equation 2-5 yields the relationship between hepatic clearance and Clint:

The relationship between intrinsic clearance and extraction ratio is shown in Figure 2-12, calculated at a hepatic blood flow of 1,400 mL/min. In general, true hepatic clearance and extraction ratio are more useful concepts for anesthetic drugs than the intrinsic clearance. However, intrinsic clearance is introduced here because it is occasionally used in pharmacokinetic analyses of drugs used during anesthesia.

So far, we have focused on linear pharmacokinetics, that is, the pharmacokinetics of drugs whose metabolic rate at clinical doses is less than Vm/3. The clearance of such drugs is generally expressed as a constant (e.g., propofol clearance = 1.6 L per minute). Some drugs, such as phenytoin, exhibit saturable pharmacokinetics (i.e., have such low Vm that typical doses exceed the linear portion of Figure 2-9). The clearance of drugs with saturable metabolism is a function of drug concentration, rather than a constant.
Renal Clearance
Renal excretion of drugs involves (a) glomerular filtration, (b) active tubular secretion, and (c) passive tubular reabsorption. The amount of drug that enters the renal tubular lumen depends on the fraction of drug bound to protein and the glomerular filtration rate (GFR). Renal tubular secretion involves active transport processes, which may be selective for certain drugs and metabolites, including protein-bound compounds. Reabsorption from renal tubules removes drug that has entered tubules by glomerular filtration and tubular secretion. This reabsorption is most prominent for lipid-soluble drugs that can easily cross cell membranes of renal tubular epithelial cells to enter pericapillary fluid. Indeed, a highly lipid-soluble drug, such as thiopental, is almost completely reabsorbed such that little or no unchanged drug is excreted in the urine. Conversely, production of less lipid-soluble metabolites limits renal tubule reabsorption and facilitates excretion in the urine.
The rate of reabsorption from renal tubules is influenced by factors such as pH and rate of renal tubular urine flow. Passive reabsorption of weak bases and acids is altered by urine pH, which influences the fraction of drug that exists in the ionized form. For example, weak acids are excreted more rapidly in alkaline urine. This occurs because alkalinization of the urine results in more ionized drug that cannot easily cross renal tubular epithelial cells, resulting in less passive reabsorption.
Renal blood flow is inversely correlated with age, as is creatinine clearance, which is closely related to GFR because creatinine is water soluble and not resorbed in the tubules. Creatinine clearance can be predicted from age and weight according to the equation of Cockroft and Gault9:
Men:

Women:
85% of the aforementioned equation.
Equation 2-7 shows that age is an independent predictor of creatinine clearance. Elderly patients with normal serum creatinine have about half the GFR than younger patients. This can be seen graphically in Figure 2-13.

Absorption
Classically, pharmacokinetics is taught as “absorption, distribution, metabolism, and elimination.” Because most anesthetic drugs are administered intravenously and inhaled anesthetic pharmacokinetics are discussed elsewhere, this order has been changed in this textbook to put absorption at the end of the list. Absorption is simply not particularly relevant for most anesthetic drugs.
Ionization
Most drugs are weak acids or bases that are present in solutions in ionized and nonionized form. The nonionized molecule is usually lipid soluble and can diffuse across cell membranes including the blood–brain barrier, renal tubular epithelium, gastrointestinal epithelium, placenta, and hepatocytes (Table 2-1). As a result, it is usually the nonionized form of the drug that is pharmacologically active, undergoes reabsorption across renal tubules, is absorbed from the gastrointestinal tract, and is susceptible to hepatic metabolism. Conversely, the ionized fraction is poorly lipid soluble and cannot penetrate lipid cell membranes easily (see Table 2-1). A high degree of ionization thus impairs absorption of drug from the gastrointestinal tract, limits access to drug-metabolizing enzymes in the hepatocytes, and facilitates excretion of unchanged drug, as reabsorption across the renal tubular epithelium is unlikely.

Determinants of Degree of Ionization
The degree of drug ionization is a function of its dissociation constant (pK) and the pH of the surrounding fluid. When the pK and the pH are identical, 50% of the drug exists in both the ionized and nonionized form. Small changes in pH can result in large changes in the extent of ionization, especially if the pH and pK values are similar. Acidic drugs, such as barbiturates, tend to be highly ionized at an alkaline pH, whereas basic drugs, such as opioids and local anesthetics, are highly ionized at an acid pH. Acidic drugs are usually supplied in a basic solution to make them more soluble in water and basic drugs are usually supplied in an acidic solution for the same reason, unless the pH affects drug stability, as is the case for most ester local anesthetics.
Ion Trapping
Because it is the nonionized drug that equilibrates across lipid membranes, a concentration difference of total drug can develop on two sides of a membrane that separates fluids with different pHs,10 because the ionized concentrations will reflect the local equilibration between ionized and nonionized forms based on the pH. This is an important consideration because one fraction of the drug may be more pharmacologically active than the other fraction.
Systemic administration of a weak base, such as an opioid, can result in accumulation of ionized drug (ion trapping) in the acid environment of the stomach. A similar phenomenon occurs in the transfer of basic drugs, such as local anesthetics, across the placenta from mother to fetus because the fetal pH is lower than maternal pH. The lipid-soluble nonionized fraction of local anesthetic crosses the placenta and is converted to the poorly lipid-soluble ionized fraction in the more acidic environment of the fetus. The ionized fraction in the fetus cannot easily cross the placenta to the maternal circulation and thus is effectively trapped in the fetus. At the same time, conversion of the nonionized to ionized fraction maintains a gradient for continued passage of local anesthetic into the fetus. The resulting accumulation of local anesthetic in the fetus is accentuated by the acidosis that accompanies fetal distress. The kidneys are the most important organs for the elimination of unchanged drugs or their metabolites. Water-soluble compounds are excreted more efficiently by the kidneys than are compounds with high lipid solubility. This emphasizes the important role of metabolism in converting lipid-soluble drugs to water-soluble metabolites. Drug elimination by the kidneys is correlated with endogenous creatinine clearance or serum creatinine concentration. The magnitude of change in these indices provides an estimate of the necessary change adjustment in drug dosage. Although age and many diseases are associated with a decrease in creatinine clearance and requirement for decreased dosing, pregnancy is associated with an increase in creatinine clearance and higher dose requirements for some drugs.
Route of Administration and Systemic Absorption of Drugs
The choice of route of administration for a drug should be determined by factors that influence the systemic absorption of drugs. The systemic absorption rate of a drug determines the magnitude of the drug effect and duration of action. Changes in the systemic absorption rate may necessitate an adjustment in the dose or time interval between repeated drug doses.
Systemic absorption, regardless of the route of drug administration, depends on the drug’s solubility. Local conditions at the site of absorption alter solubility, particularly in the gastrointestinal tract. Blood flow to the site of absorption is also important in the rapidity of absorption. For example, increased blood flow evoked by rubbing or applying heat at the subcutaneous or intramuscular injection site enhances systemic absorption, whereas decreased blood flow due to vasoconstriction impedes drug absorption. Finally, the area of the absorbing surface available for drug absorption is an important determinant of drug entry into the circulation.
Oral Administration
Oral administration of a drug is often the most convenient and economic route of administration. Disadvantages of the oral route include (a) emesis caused by irritation of the gastrointestinal mucosa by the drug, (b) destruction of the drug by digestive enzymes or acidic gastric fluid, and (c) irregularities in absorption in the presence of food or other drugs. Furthermore, drugs may be metabolized by enzymes or bacteria in the gastrointestinal tract before systemic absorption can occur.
With oral administration, the onset of drug effect is largely determined by the rate and extent of absorption from the gastrointestinal tract. The principal site of drug absorption after oral administration is the small intestine due to the large surface area of this portion of the gastrointestinal tract. Changes in the pH of gastrointestinal fluid that favor the presence of a drug in its nonionized (lipid-soluble) fraction thus favor systemic absorption. Drugs that exist as weak acids (such as aspirin) become highly ionized in the alkaline environment of the small intestine, but absorption is still great because of the large surface area. Furthermore, absorption also occurs in the stomach, where the fluid is acidic.
First-Pass Hepatic Effect
Drugs absorbed from the gastrointestinal tract enter the portal venous blood and thus pass through the liver before entering the systemic circulation for delivery to tissue receptors. This is known as the first-pass hepatic effect. For drugs that undergo extensive hepatic extraction and metabolism (propranolol, lidocaine), it is the reason for large differences in the pharmacologic effect between oral and intravenous doses.
Oral Transmucosal Administration
The sublingual or buccal route of administration permits a rapid onset of drug effect because this blood bypasses the liver and thus prevents the first-pass hepatic effect on the initial plasma concentration of drug. Venous drainage from the sublingual area is into the superior vena cava. Evidence of the value of bypassing the first-pass hepatic effect is the efficacy of sublingual nitroglycerin. Conversely, oral administration of nitroglycerin is ineffective because extensive first-pass hepatic metabolism prevents establishment of a therapeutic plasma concentration. Buccal administration is an alternative to sublingual placement of a drug; it is better tolerated and less likely to stimulate salivation. The nasal mucosa also provides an effective absorption surface for certain drugs.
Transdermal Administration
Transdermal administration of drugs provides sustained therapeutic plasma concentrations of the drug and decreases the likelihood of loss of therapeutic efficacy due to peaks and valleys associated with conventional intermittent drug injections. This route of administration is devoid of the complexity of continuous infusion techniques, and the low incidence of side effects (because of the small doses used) contributes to high patient compliance. Characteristics of drugs that favor predictable transdermal absorption include (a) combined water and lipid solubility, (b) molecular weight of <1,000, (c) pH 5 to 9 in a saturated aqueous solution, (d) absence of histamine-releasing effects, and (e) daily dose requirements of <10 mg. Scopolamine, fentanyl, clonidine, estrogen, progesterone, and nitroglycerin are drugs available in transdermal delivery systems. Unfortunately, sustained plasma concentrations provided by transdermal absorption of scopolamine and nitroglycerin may result in tolerance and loss of therapeutic effect.
It is likely that transdermal absorption of drugs initially occurs along sweat ducts and hair follicles that function as diffusion shunts. The rate-limiting step in transdermal absorption of drugs is diffusion across the stratum corneum of the epidermis. Differences in the thickness and chemistry of the stratum corneum are reflected in the skin’s permeability. For example, skin may be 10 to 20 µm thick on the back and abdomen compared with 400 to 600 µm on the palmar surfaces of the hands. Likewise, skin permeation studies have shown substantial regional differences for systemic absorption of scopolamine. The postauricular zone, because of its thin epidermal layer and somewhat higher temperature, is the only area that is sufficiently permeable for predictable and sustained absorption of scopolamine. The stratum corneum sloughs and regenerates at a rate that makes 7 days of adhesion the duration limit for one application of a transdermal system. Contact dermatitis at the site of transdermal patch applications occurs in a significant number of patients.
Rectal Administration
Drugs administered into the proximal rectum are absorbed into the superior hemorrhoidal veins and subsequently transported via the portal venous system to the liver (first-pass hepatic effect), where they are exposed to metabolism before entering the systemic circulation. On the other hand, drugs absorbed from a low rectal administration site reach the systemic circulation without first passing through the liver. These factors, in large part, explain the unpredictable responses that follow rectal administration of drugs. Furthermore, drugs may cause irritation of the rectal mucosa.
Pharmacokinetic Models
In the following section, several common, useful pharmacokinetic models are derived. Although it is not necessary for every clinician to be able to derive these models, a consideration of where they come from takes them out of the “black box” and allows consideration of their representative parts.
Zero- and First-Order Processes
The consumption of oxygen and production of carbon dioxide are processes that happen at a constant rate. These are called zero-order processes. The rate of change (dx/dt) for a zero-order process is . This says the rate of change is constant. If x represents an amount of drug and t represents time, then the units of k are amount/time. If we want to know the value of x at time t, x(t), we can compute it as the integral of this equation from time 0 to time t, x(t) = x0 + k · t, where x0 is the value of x at time 0. This is the equation of a straight line with a slope of k and an intercept of x0.
Many processes occur at a rate proportional to the amount. For example, the interest payment on a loan is proportional to the outstanding balance. The rate at which water drains from a bathtub is proportional to amount (height) of water in the tub. These are examples of first-order processes. The rate of change in a first-order process is only slightly more complex than for a zero-order process, . In this equation, x has units of amount already, so the units of k are 1/time. The value of x at time t, x(t), can be computed as the integral from time 0 to time t, x(t) = x0 ekt, where x0 is the value of x at time 0. If k > 0, x(t) increases exponentially to infinity. If k < 0, x(t) decreases exponentially to 0. In pharmacokinetics, k is negative because concentrations decrease over time. For clarity, the minus sign is usually explicit, so k is expressed as a positive number. Thus, the identical equation for pharmacokinetics, with the minus sign explicitly written, is

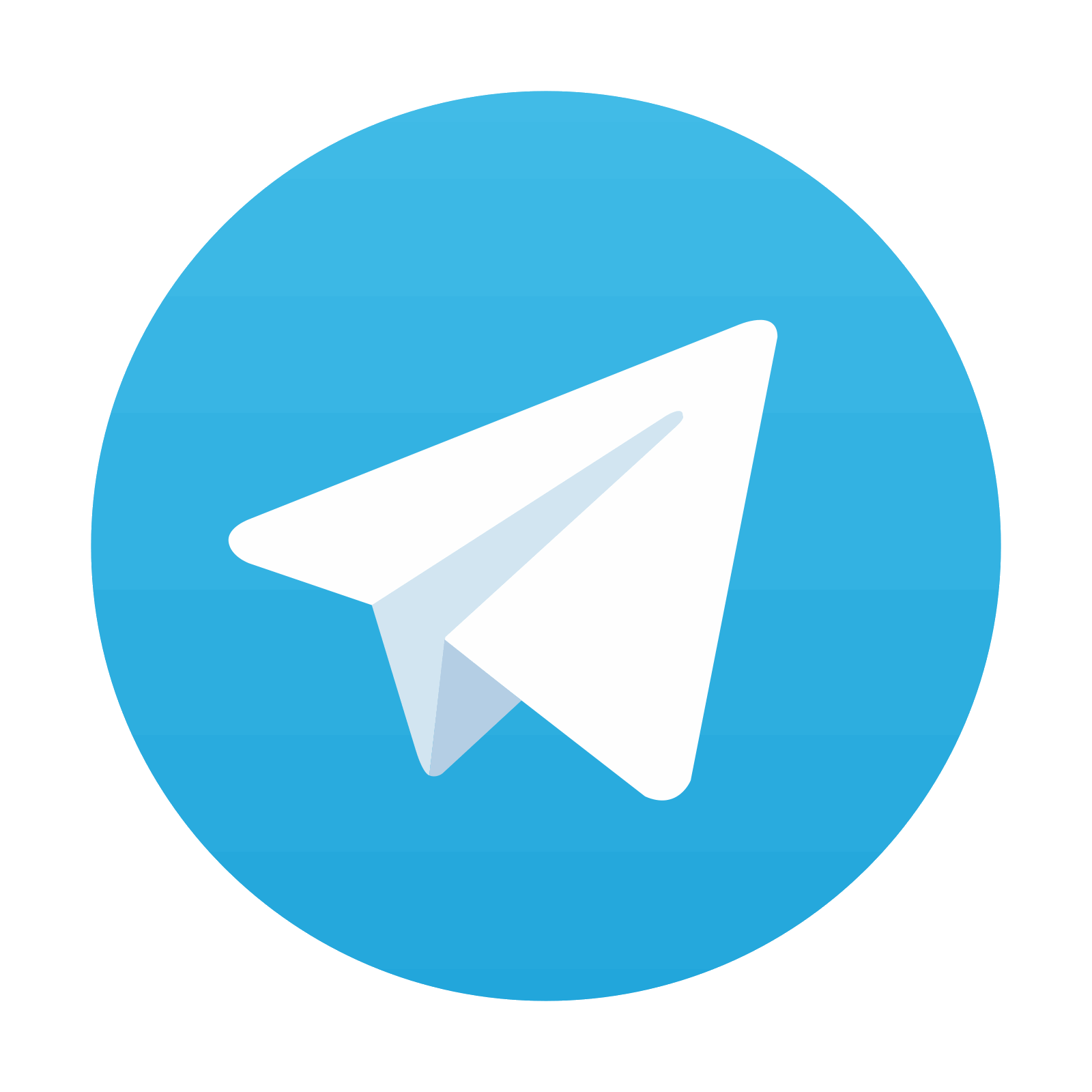
Stay updated, free articles. Join our Telegram channel
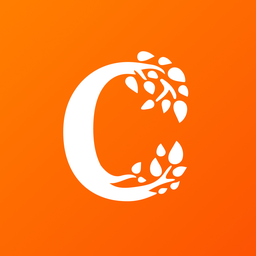
Full access? Get Clinical Tree
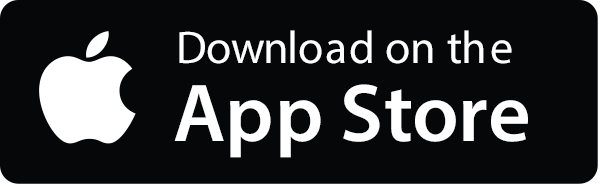
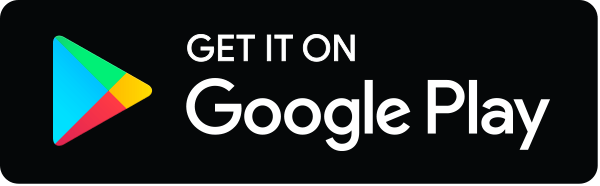