(1)
Division of Pulmonary and Critical Care Medicine, Eastern Virginia Medical School, Norfolk, VA, USA
Keywords
Acute respiratory distress syndrome (ARDS)Mechanical ventilationTidal volumeAcute Respiratory Distress Syndrome Network (ARDSnet)Prone positioningPositive end-expiratory pressurePressure-volume curveAcute lung injurySepsisExtravascular lung water index (EVLWI)Inhaled nitric oxide (iNO)Plateau airway pressureBarotraumaAlveolar overdistensionPressure controlled ventilation (PCV)Airway pressure release ventilation (APRV)Esophageal tonometryPermissive hypercapniaRecruitment maneuverExtracorporeal Membrane Oxygenation(ECMO)CorticosteroidsIf we are to survive, we must have ideas, vision, and courage. These things are rarely produced by committees. Everything that matters in our intellectual and moral life begins with an individual confronting his own mind and conscience in a room by himself.
Arthur M. Schlesinger, Jr, American Historian (1917–2007).
Definition, Causes and Assessment of Severity
The adult respiratory distress syndrome (ARDS) was initially described by Ashbaugh and Petty as a syndrome characterized by diffuse pulmonary infiltrates, with decreased pulmonary compliance and hypoxemia [1]. It has however been recognized that “ARDS” is a spectrum varying from mild acute lung injury (ALI) at one end to ARDS at the other. The diagnosis of ARDS should be reserved for patients with ALI who have severe disease (see criteria below). The outcome of ALI is largely dependent on both the severity of ALI and the causative factors. It should be emphasized that in most cases ALI is a multi-system disease; the microcirculatory changes which occur in the lung occur in all organs; the pathophysiological derangements however, are most evident in the lung.
Definition of ALI According the American European Consensus [2]
A condition involving:
an oxygenation defect with bilateral alveolar infiltrates,
a patient who has suffered an acute catastrophic event,
who has a pulmonary capillary wedge pressure ≤18 mmHg or no clinical evidence of an elevated left atrial pressure.
Acute Lung Injury (ALI)
A patient is defined as having ALI when the PO2/FiO2 ≤300 (regardless of the amount of PEEP).
Acute Respiratory Distress Syndrome (ARDS)
A patient is said to have ARDS when the PO2/FiO2 ≤200 (regardless of the amount of PEEP).
In 2012 the ARDS Task Force published the “Berlin Definition of Acute Respiratory Distress Syndrome.” (See Table 23.1) [3] This definition seems to add little to American European Consensus Definition published in 1994. However it seems that if you have nothing better to do, you assemble a tasks force of your own “co-conspirators”, develop a new definition/or guideline which you must then publish.
Criteria | Definition |
---|---|
Timing | Within 1 week of known clinical insult or new or worse respiratory symptoms |
Chest Imaging | Bilateral opacities- not fully explained by effusions, lobar/lung collapse or nodules |
Origin of edema | Resp. failure not explained by cardiac failure or fluid overload. Need objective assessment (ECHO) to exclude hydrostatic edema |
Oxygenation | |
Mild | PaO2/FiO2 between 200 and 300 with PEEP ≥5 cm H2O |
Moderate | PaO2/FiO2 between 100 and 200 with PEEP ≥5 cm H2O |
Severe | PaO2/FiO2< 100 with PEEP ≥5 cm H2O |
Pathophysiological Definition of ARDS
The typical pathological feature of ARDS is diffuse alveolar damage (DAD), which result in interstitial and alveolar edema and accumulation of extravascular lung water (EVLW). Since it is possible to accurately measure EVLW (see transpulmonary thermo-dilution, Chap. 10) this would appear to be the most precise method to diagnose and quantitate the severity of ARDS. The normal EVLW value has been shown to be approximately 7 ± 3 mL/kg [4]. Furthermore, transpulmonary thermo-dilution can accurately distinguish between cardiogenic and non-cardiogenic pulmonary edema.
Tagami et al. compared the postmortem weights of normal lungs with those from patients with diffuse alveolar damage [5]. These lung weights were converted to extravascular lung water (EVLW) values using a validated equation. The extravascular lung water value that indicated diffuse alveolar damage was estimated using receiver operating characteristic analysis. The EVLW of the lungs showing diffuse alveolar damage were approximately twofold higher than those of normal lungs (normal group, 7.3 ± 2.8 mL/kg vs diffuse alveolar damage group 13.7 ± 4.5 mL/kg; p < 0.001). An EVLW of >9.8 mL/kg had an area under the ROC curve of 0.90 (CI, 0.88–0.91) for the diagnosis of ALI. Furthermore, EVLW has been demonstrated to be highly predictive of outcome with an EVLW >16 mL/kg being associated with a very high mortality [6, 7].
Causes of ALI [8, 9]
ALI may result from either direct or indirect lung injury. It is likely that the severity of ALI and the outcome is related to the causation of ALI. The common causes include:
Direct lung injury
pneumonia
aspiration pneumonitis
smoke inhalation
chemical inhalation
drowning
Indirect lung injury
sepsis and sepsis syndrome
poly-trauma
Transfusion of blood and blood products (TRALI)
pancreatitis
drug induced (heroin, tricyclic antidepressants, etc.)
fat embolism
burns
Management of the Acute Phase of ARDS
The management of ARDS is essentially supportive; cardio-respiratory and nutritional support, the prevention of further lung injury and the prevention of complications while waiting for the acute inflammatory response to resolve and lung function to improve [9–11]. Tonelli et al. performed an umbrella review of 159 published randomized trials and 29 meta-analyses which evaluated the outcome of specific interventions in ARDS [12]. The authors concluded that there was only consistent evidence for low tidal volume ventilation and prone positioning in severe ARDS.
Ventilatory Strategy
The most important “recent” advance in the management of patients with ARDS (indeed in critical care medicine) is the realization that overdistension of alveoli causes acute lung injury. Hence a “lung protective strategy” is the standard of care and the cornerstone of the management of patients with ARDS [13]. Tidal volumes (Vt) should not exceed 6 mL/kg PBW (see Chap. 19).
The chest radiographs of patients with ARDS classically show widespread involvement of all lung fields. It was therefore assumed that ARDS was a homogenous process. However, high resolution computed tomographic scans performed in patients with ARDS have demonstrated areas of normal, consolidated and overinflated lung. The large area of consolidated and collapsed lung is predominantly distributed in the dependent areas, and participates minimally in gas exchange. The normal lung is usually anterior and often markedly overdistended. In addition, in the early stages of ARDS, consolidated lung units can be “recruited” with the application of modest distending pressures. Consequently, patients with ARDS typically have three functionally distinct lung zones; namely;
that portion of the lung that is diseased and not recruitable,
that portion of the lung that is diseased but recruitable and
that portion of the lung that is normal.
Because a significant portion of the lung is consolidated and not recruitable, only a small amount of aerated lung receives the total tidal volume—ARDS leads to “baby lungs” [14]. The use of “traditional” tidal volumes (12 mL/kg) in these patients will result in high inspiratory pressures with overdistension of the normally aerated lung units. A growing body of experimental evidence has demonstrated that mechanical ventilation that results in high trans-pulmonary pressure gradients and overdistension of lung units will cause acute lung injury, characterized by hyaline membranes, granulocytic infiltration, pulmonary hypertension, and increased pulmonary and systemic vascular permeability. Animal studies have demonstrated that a trans-pulmonary pressure in excess of 35 cm H2O will lead to alveolar damage [15]. These studies have demonstrated that ventilation with low tidal volumes preserves pulmonary ultrastructure. Furthermore, it has been postulated that the cyclic opening and closing of lung units (recruitment and derecruitment) in patients with ARDS who are ventilated with insufficient PEEP may further potentiate this iatrogenic lung injury [8, 9, 16]. It has therefore been suggested that ventilatory strategies that avoid regional or global overdistension of lung units and also avoids end-expiratory alveolar collapse may limit the degree of lung injury in ARDS…the open lung approach [17].
The Acute Respiratory Distress Syndrome Network randomized patients with ARDS to receive traditional volume controlled ventilation (an initial tidal volume of 12 mL/kg and an plateau pressure of ≤50 cm of water) or low tidal volume ventilation (an initial tidal volume of 6 mL/kg and a plateau pressure of ≤30 cm of water) [13]. In the low Vt group, Vt was reduced further to 5 or 4 mL/kg PBW if necessary to maintain plateau pressure (Pplat) at less than 30 cm H2O. The trial was stopped after the enrollment of 861 patients because mortality was lower in the group treated with lower tidal volumes (31.0 % vs. 39.8 %, p = 0.007). This study has provided convincing evidence that a strategy that avoids alveolar overdistension in ARDS improves outcome.
The response to low-tidal-volume ventilation should be assessed initially on the basis of plateau airway pressure. The goal should be to maintain a plateau airway pressure (i.e., the pressure during an end-inspiratory pause) of 30 cm of water or less; if this target is exceeded, the tidal volume should be further reduced to a minimum of 4 mL per kilogram of predicted body weight. An important caveat relates to patients who have stiff chest walls (for example, those with massive ascites or morbid obesity). In such patients, it is reasonable to allow the plateau pressure to increase to values greater than 30 cm of water, since the pleural pressures are elevated and hence the transpulmonary pressures are not elevated (i.e., there is not necessarily alveolar overdistention). Ideally in these patients ventilatory management is guided by placing an esophageal balloon and adjusting the Tv and PEEP such that one avoids a high transpulmonary pressure at end-expiration (<25 cm H2O) and thereby avoiding alveolar overdistension while adjusting PEEP such that the transpulmonary pressure is greater than 0 cm H2O at end-expiration (0–5 cm H2O) to avoid alveolar derecruitment thereby preventing repetitive alveolar collapse and reopening (aletectrauma). Talmor and colleagues performed a randomized controlled study in which PEEP and Vt were set according to measurement of esophageal pressures or according to the ARDSNet protocol [18]. In this pilot study, oxygenation and respiratory compliance were significantly better in the esophageal pressure group with a trend towards improved survival.
The available data does not support the commonly held view that inspiratory plateau pressures of 30–35 cm H2O are safe [19]. There is no safe upper limit for plateau pressures in patients with ALI/ARDS. The lower the plateau pressure the lower the mortality (see Fig. 23.1); i.e. a Vt of 6 mL/kg/PBW should be used even if the plateau pressures are less than 28 cm H2O.
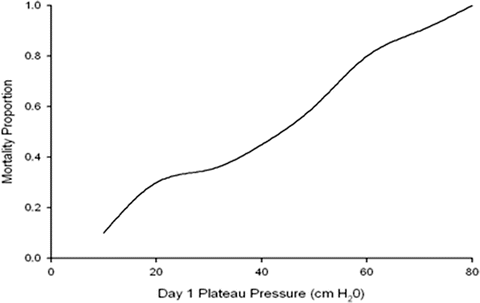
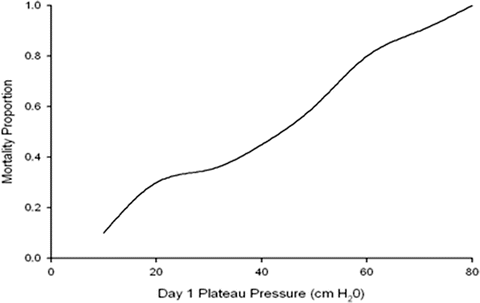
Fig. 23.1
Relationship between mortality and plateau pressure
A number of authors have suggested that a low-tidal volume ventilatory strategy is cardio protective rather than lung protective. Jardin and Vieillard-Baron have demonstrated a progressive increase in the incidence of acute cor pulmonale as the plateau pressure increases [20]. In their study the mortality rate and incidence of acute cor pulmonale increased markedly in ARDS patients above a plateau of 26 cm H2O (see Fig. 23.2).
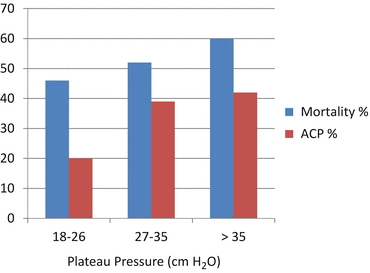
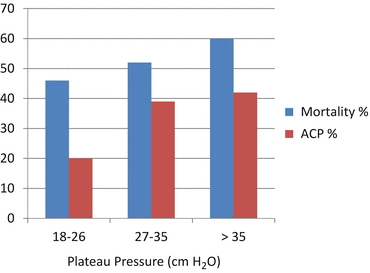
Fig. 23.2
Mortality and incidence of acute cor pulmonale (ACP) plotted against plateau pressure. Adapted from Jardin and Vieillard-Baron [20]
While sepsis and multi-system organ failure (MSOF) remain the most common cause of death in patients with ARDS up to 20 % of deaths are attributable to progressive respiratory failure [21]. A number of interventions have been attempted in this group of patients including inhaled nitric oxide, nebulized prostacyclin and surfactant, recruitment maneuvers, liquid ventilation, high frequency oscillation and prone positioning. With the exception of prone positioning in patients with severe ARDS (see below) there is little evidence that these interventions improve outcome [9–11, 22].
Pressure controlled ventilation (PCV) and Airway Pressure Release Ventilation (APRV) have been used in patients with refractory hypoxemia ventilated with a low-tidal volume ventilatory strategy. APRV has emerged as an alternative ventilatory strategy in patients with severe ARDS (see Chap. 19) [23–25]. PCV and APRV have however, yet to be carefully compared with volume-cycled ventilation in patients with ARDS in terms of morbidity, length of mechanical ventilation and ultimate patient outcome in a RCT. It is unlikely that such a trial will be performed; however, from the forgoing it is likely that ventilation strategies that achieve the same end-points (i.e. prevent alveolar overdistension and limit airway pressures) will have similar outcomes.
High frequency oscillation has been used as a rescue ventilatory strategy in patients with ARDS and refractory hypoxemia. High-frequency oscillatory ventilation (HFOV) delivers very small tidal volumes (approximately 1 to 2 mL/kg) at very high rates (3–15 breaths per second) and is considered a true lung protective ventilatory strategy. HFOV combines small pressure oscillations to minimize overdistension with high mean airway pressure to prevent atelectrauma. Two randomized controlled studies were reported in 2013 that failed to show a benefit of this strategy. The OSCILLATE trial randomized 548 patients with moderate-to-severe ARDS at 39 ICUs to HFOV or a control strategy with the use of low tidal volumes (mean Tv 6.1 mL/kg IBW) and high PEEP (mean 18 cm H2O) [26]. The trial was terminated prematurely due to an increase in mortality in the HFOV arm (47 % vs. 35 %, RR 1.33; CI 1.09–1.64, p = 0.005). HFOV was associated with higher mean airway pressures and with greater use of sedatives, neuromuscular blockers, and vasoactive drugs. The OSCAR trial randomized 795 patients with ARDS and a PaO2/FiO2 of less than 200 to HFOV or “usual care” in 29 hospitals in England, Wales, and Scotland [27]. Unlike the OSCILLATE trial a lung protective strategy was recommended but not mandated in the control arm (average Tv ? 8.3 mL/kg IBW). In this study there was no significant difference in mortality (41.7 vs 41.1 %) or other secondary end-points. The hemodynamic compromise associated with HFOV was minimal in the OSCAR trial as compared to the oscillate trial, perhaps owing to the lower applied ventilatory pressures in the OSCAR trial. In both trials the patients in the HFOV group received more muscle relaxants and sedatives than did patients in the control group.
Pressure Controlled Ventilation
To prevent alveolar overdistension and reduce the transpulmonary pressure gradients the inspiratory pressure is set such that the peak inspiratory pressure is less than 30 cm H2O (i.e. applied PEEP + Inspiratory Pressure <30 cm H2O) when possible, and always less than 35 cm H2O. An inspiratory pressure of 20 cm H2O (plus PEEP of 10 cm H2O) with a respiratory rate of 16 breaths/min are convenient starting points.
The inspiratory and expiratory times (or I:E ratio) and respiratory rate are best determined by analyzing the Flow vs. Time Waveform (See Fig. 23.3). Flow will initially enter the lung rapidly because the ventilator attempts to reach the set airway pressure as quickly as it can (point A, Fig. 23.3). Airways that are open and have the least resistance will receive the greatest amount of gas flow and reach equilibrium with the pre-set pressure more quickly than airways with greater resistance. As the open airways fill and the lung pressure reaches equilibrium with the pre-set pressure, flow will decelerate as the airways with higher resistance continue to fill with gas (point B, Fig. 23.3). Flow into the lung will continue until one of two events occur,
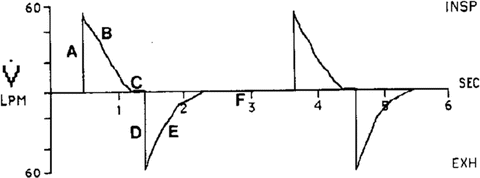
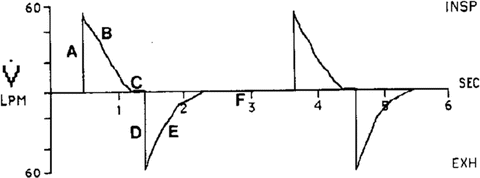
Fig. 23.3
Flow vs Time during pressure control ventilation
the preset pressure reaches equilibrium throughout all lung units (indicated by the flow pattern decelerating to zero), or
the pre-set inspiratory time ends inspiration before pressure has equilibrated throughout all lung units (indicating by the flow pattern not reaching zero).
When inspiratory flow reaches zero it means the pressure in the lung is equal to the pressure set on the ventilator (point C, Fig. 23.3). It is essential that adequate inspiratory time be given so that all the airways, both healthy and diseased, have time to reach the preset pressure level. In ARDS much of the airway bed may take a relatively long time to open. For this reason it may be necessary to lengthen the inspiratory time, sometimes to the point that the inspiratory time is longer than the expiratory time. If air trapping is not present this approach will increase mean airway pressure without increasing maximal end expiratory pressure. In patients with ARDS oxygenation is primarily a function of mean airway pressure. This strategy will therefore increase alveolar ventilation and improve oxygenation.
The inspiratory time can be lengthened in 2 ways;
if the ventilator will allow for the adjustment of inspiratory time, then simply increase the inspiratory time until the inspiratory flow reaches zero (recommended method),
if the ventilator will allow adjustment of the I/E ratio, then reducing the “E” part of the ratio will increase “I”.
If flow reaches zero and there is a long inspiratory pause, this is an indication that inspiratory time is too long. There is little benefit of having a prolonged inspiratory pause. Setting inspiratory time longer than that which is required to open recruitable airways increases the likelihood of significant auto-peep with its attendant hemodynamic complications.
To evaluate the adequacy of the expiratory time, the Flow vs. Time Waveform (Fig. 23.3) needs to be studied again. This waveform shows whether the patient has enough time to exhale to the pre-set PEEP level before the ventilator gives the next breath. In Fig. 23.3, point D represents the beginning of exhalation. When exhalation begins gas will exit the lungs quickly at first because a large pressure gradient exists between the lungs and the atmosphere. As gas continues to exit the lungs the pressure gradient will become smaller and flow will decelerate (point E, Fig. 23.3). Exhalation will continue until one of two events occur;
the pressure in the lung reaches atmospheric pressure plus the set PEEP pressure (point F, Fig. 23.3) or
the set inspiratory time mandates that inhalation begin before exhalation of the previous breath is complete thus causing auto-peep.
Figure 23.4 demonstrates gas trapping as inhalation begins before expiratory flow is allowed to reach zero. Should gas trapping be evident on the Flow vs. Time Waveform, either the respiratory rate or inspiratory time should be reduced, allowing time for complete exhalation and thereby minimizing auto-PEEP. The respiratory rate and inspiratory time should both be independently and sequentially reduced, in order to determine which maneuver affects ventilation the least.
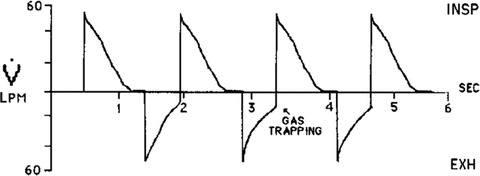
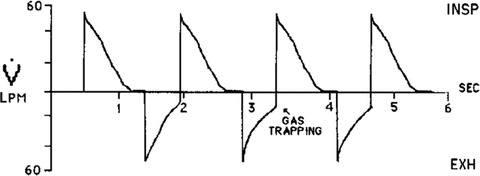
Fig. 23.4
Flow vs Time during pressure control ventilation demonstrating air trapping
It is essential that the level of auto-PEEP be measured in all patients receiving PCV. There is no data that intrinsic PEEP has any advantage over extrinsic (i.e. applied) PEEP. However, the unrecognized development of auto-PEEP may result in hemodynamic compromise leading to the inappropriate use of fluid and vasopressor therapy. The Flow vs Time waveform should be monitored regularly. As the patients pulmonary mechanics change the inspiratory time and respiratory rate may need to be altered. Once the patients’ condition has stabilized attempts should be made to reduce the level of PEEP (and FiO2).
Airway Pressure Release Ventilation
APRV was first described by Stock and colleagues in 1987, and has been commercially available since the mid 1990s [28]. APRV can be classified as a pressure-limited, time-cycled mode of mechanical ventilation that allows the patient unrestricted spontaneous breathing during the application of continuous positive airway pressure [29–31]. It is an alternative approach to the “open-lung” ventilation strategy [31]. Although recruitment maneuvers may be effective in improving gas exchange and compliance, these effects are not sustained; APRV may be viewed as a nearly continuous recruitment maneuver [29]. The ventilator maintains a high-pressure setting for the bulk of the respiratory cycle (PHigh), which is followed by a periodic release to a low pressure (PLow) [32](see Fig. 23.5). The periodic releases aid in carbon dioxide elimination (CO2). The release periods (TLow) are kept short (0.7–1 s); this prevents derecruitment and enhances spontaneous breathing during THigh [31, 33]. The release volumes must be monitored during APRV and should be kept below 8 mL/kg/ IBW to prevent alveolar overdistension. The advantages of APRV over volume controlled ventilation include an increase in mean alveolar pressure with alveolar recruitment, the hemodynamic and ventilatory benefits associated with spontaneous breathing and the reduced requirement for sedation.
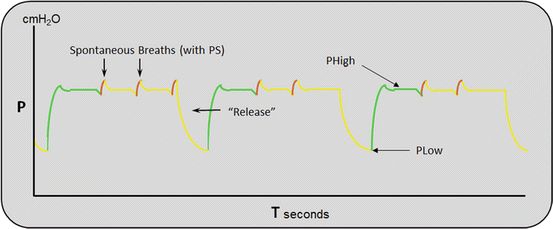
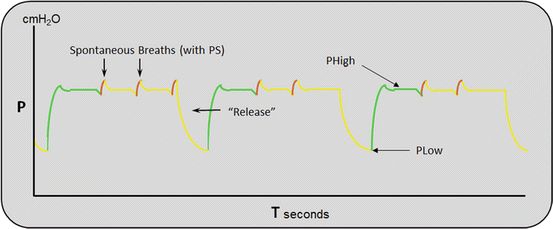
Fig. 23.5
Pressure time waveform during APRV
APRV uses an active exhalation valve that allows spontaneous breathing throughout the respiratory cycle. Due to the short release time (TLow) the spontaneous breaths occur almost exclusively during the PHigh [31, 33]. Both experimental and clinical studies have demonstrated that the addition of spontaneous breaths to APRV recruits dependent lung regions, increases end-expiratory lung volume, decreases V/Q mismatching, and improves oxygenation, cardiac function (cardiac index) and organ blood flow [34–40].
Lung protective strategies using both volume and pressure controlled ventilation are usually poorly tolerated requiring deep sedation. APRV however, is extremely well tolerated by patients allowing sedation to be reduced and even discontinued in many patients. This is a very important issue as the increased use of sedation has been associated with a longer duration of mechanical ventilation as well as an increased incidence of ventilator associated pneumonia, delirium and an increased mortality.
We have previously demonstrated a significant improvement in oxygenation with decreased V/Q mismatching (increased PaO2/FiO2 and decreased Vd/Vt) in a cohort of patients with severe ARDS who were switched from volume-controlled ventilation to APRV [41]. Similarly, improved oxygenation and hemodynamic parameters with APRV have been demonstrated in other observational studies [25, 39, 40, 42–44]. Andrews et al. compared the outcomes reported in the literature of patients with acute traumatic injuries that were ventilated using conventional ventilation with their experience in which APRV is used as the default mode of ventilation [45]. In this uncontrolled comparative observational study the early use of APRV was associated with a lower incidence of ARDS (14.0 % vs. 1.3 %) and in-hospital mortality (14.1 % vs. 3.9 %). These authors postulated that the early use of APRV may prevent progression of acute lung injury in high-risk trauma. A limitation of this study is that it is likely that a preventative lung protective strategy was not adopted in the control trauma centers, as it has been now well established that “high” tidal volumes will cause ALI in perviously normal lungs.
It is important to emphasize that a ventilatory strategy that results in an improvement in oxygenation may not translate into an improvement in patient outcome (may even be worse). Since APRV has not been compared to conventional low tidal volume ventilation in a RCT, this strategy should only be considered in patients who have “failed” the conventional low tidal volume lung protective strategy. Currently a number of (small) RCTs are being conducted comparing conventional low tidal volume ventilation with APRV; these studies should provide additional information on the value of this ventilatory mode (NCT01339533, NCT01901354, and NCT00793013).
Permissive Hypercapnia
The strategy to reduce volume induced lung injury by using small tidal volumes may lead to CO2 retention. The term “permissive hypercapnia” has been used to describe this ventilatory strategy. Hypercapnic acidosis is generally well tolerated by the patients, especially when it develops gradually over 1 to 2 days. The intracellular acidosis is corrected rapidly during sustained hypercapnia, whereas the extracellular acidosis may persist for much longer. The lowest pH that can be safely tolerated in unknown, however, a pH greater than 7.2 is generally recommended. Some patients, however, have tolerated a pH as low as 7.05 without obvious adverse effects. It has been suggested that bicarbonate should be used to correct the pH. However, the administration of bicarbonate may paradoxically increase intracellular acidosis. Permissive hypercapnia should not be used in patients with acute intracranial pathology as this may cause a precipitous increase in intracranial pressure. Furthermore in patients with ischemic heart disease, arrhythmias and patients requiring high doses of inotropic drugs, hypercapnia should be allowed to develop gradually. Surprisingly, permissive hypercapnia itself has anti-inflammatory effects and has been shown to attenuate lung injury in animal models [46, 47]. Furthermore, permissive hypercapnia has beneficial hemodynamic effects [47].
Best PEEP
Positive end-expiratory pressure (PEEP) appears to be protective against ventilator-induced lung injury in animal studies, perhaps by recruiting more aerated lung and preventing shear forces produced during repetitive opening of closed airways or alveoli. Low tidal volume ventilation has been demonstrated to cause a decline in compliance in healthy subject as well as patients in respiratory failure. It has been suggested that the smaller the tidal volume the higher the PEEP level need to optimize lung mechanics. It is generally believed that PEEP set below 10 cm H2O will probably keep healthy alveoli open at end exhalation, but will not be enough to distend diseased airways. These airways will then continually open and collapse throughout the ventilator cycle. The goal is to set PEEP at a level that does not overdistend healthy alveoli but at the same time does not let diseased airways collapse. The term the “Open Lung Approach” has been used to describe this method of ventilation [17]. It has been reported that in patients with ARDS a mean PEEP level of 15 cm H2O is required to keep the airways “open” at end-expiration [17].
While the beneficial effects of a low tidal volume strategy is largely accepted, the role of PEEP as part of the “Lung Protective Strategy” is more controversial [48–50]. A meta-analysis demonstrated a trend towards improved mortality with high PEEP, even though the difference did not reach statistical significance; with the pooled cumulative risk of 0.90 (95 % CI 0.72–1.02, P = 0.077) [51]. The reduction in absolute risk of death was approximately 4 %. There was no evidence of a significant increase in baro-trauma in patients receiving high PEEP, with a pooled risk of 0.95 (95 % CI 0.62–1.45, P = 0.81).
“Best PEEP” can be estimated from a static/dynamic pressure/volume curve (see Fig. 23.6). This curve classically demonstrates an upper and lower inflection point. PEEP should be set above the lower inflection point such that the sum of the PEEP and the inspiratory pressure should be below 30 cm H2O (a plateau pressure up to 35 may be acceptable) or the upper inflection point. Should an inflection point not be present on the pressure/volume curve or it not be possible to perform this maneuver, the initial PEEP should be set between 10 and 15 cm H2O. Alternate methods of setting PEEP include setting PEEP above the point of derecruitment on the expiratory limb of the dynamic pressure volume curve (see Fig. 23.6) or by measuring transpleural pressures with an esophageal balloon (as discussed previously).
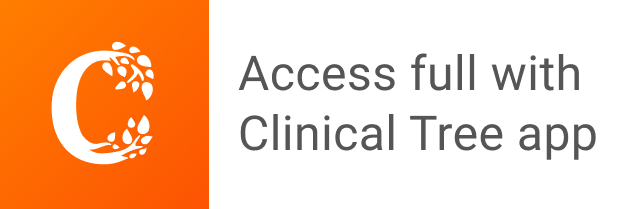