Chapter Outline
Normal Platelet Function and Hemostasis
Mechanisms of Thrombin and Fibrin Generation
Indirect Inhibitors of Thrombin Generation
Arterial and venous thrombosis are major causes of morbidity and mortality. Venous thromboembolism (VTE) is the leading cause of preventable in-hospital mortality. Deep vein thrombosis (DVT) leading to VTE causes as many as 300,000 deaths annually in the United States and approximately 300,000 within the European Union as well.
Arterial thrombosis is the most common cause of myocardial infarction (MI), ischemic stroke, and limb gangrene. Arterial thrombi, which typically form under high shear conditions, consist of platelet aggregates held together by small amounts of fibrin. Because of the predominance of platelets, strategies to inhibit arterial thrombogenesis focus mainly on drugs that block platelet function, but include anticoagulants for prevention of cardioembolic events in patients with atrial fibrillation or mechanical heart valves.
DVT can lead to pulmonary thromboembolism (PE), which can be fatal, and to postphlebitic syndrome. Venous thrombi, which form under low shear, are composed mainly of fibrin and trapped red blood cells, with relatively few platelets. With the predominance of fibrin in venous thrombi, anticoagulants are the agents of choice for the prevention and treatment of DVT and VTE.
The incidence of VTE is about 20% higher in men than in women and increases with age in both sexes. Asian and Hispanic populations have a lower risk of VTE, whereas Caucasians and African Americans have a 2.5-fold higher risk.
Agents such as vitamin K antagonists or dual antiplatelet therapy with aspirin and clopidogrel have addressed inappropriate thrombosis. Administration of these drugs significantly reduces the risk of thrombotic events but also increases bleeding. They also suffer from inconsistency in efficacy associated with pharmacogenetic factors and drug interactions. Recently there have been a number of additional anticoagulant and antiplatelet drugs based on a greater understanding of hemostasis mechanisms that are outlined in the following text.
Normal Platelet Function and Hemostasis
A brief overview of the hemostatic system is provided as background for consideration of antiplatelet and anticoagulant drugs; further detail is provided in Chapters 43 and 44 . The initial response of the hemostatic system to tissue or endothelial injury is to produce a platelet plug (primary hemostasis). Platelets have multiple surface receptors that, when stimulated, produce a shape change involving the energy-dependent actin-myosin system. Principal among these is the glycoprotein Ib (GpIb) receptor, which binds to von Willebrand factor (vWF), a large protein expressed on the abluminal side of endothelial cells that is exposed when there is endothelial injury. The glycoprotein Ib receptor is expressed at all times. There are also receptors for adenosine diphosphate (ADP), thrombin, and thromboxane A 2 . These receptors principally allow feedback amplification to enhance generation of the primary hemostatic plug.
With the platelet shape change, the surface of the platelet also changes with expression of a second binding site, the glycoprotein IIb/IIIa (GpIIb/IIIa) receptor. The GpIIb/IIIa receptor binds fibrinogen to provide bridging between adjacent platelets. The surface of the platelet also becomes electronegatively charged and expresses binding sites for factor V, an essential cofactor in the generation of thrombin.
Mechanisms of Thrombin and Fibrin Generation
The coagulation phase of hemostasis involves thrombin-catalyzed cleavage of fibrinogen to fibrin, which binds and stabilizes the weak platelet hemostatic plug. Thrombin is highly specific in cleaving fibrinogen at only two arginine sites. The active site of thrombin is surrounded by negatively charged amino acid residues and away from this are positively charged exosites. This arrangement allows the very specific alignment and cleavage of fibrinogen ( Fig. 45.1 ).

There are no covalent bonds holding platelets together during formation of the primary hemostatic plug. If left in this state, the platelet plug disintegrates in a few hours, resulting in late bleeding. The process of blood coagulation, with soluble factors in the blood entering into a cascade of protease activation that leads to the formation of fibrin, is localized to the site where the original platelet plug was formed. This localization is achieved by two mechanisms. First, the chain of reactions that leads to cleavage of fibrinogen to fibrin is restricted to a surface, such as platelet phospholipids. Second, a series of inhibitors constrains the reaction to the site of injury and platelet deposition.
Historically the blood coagulation system is separated into two initiating pathways: the tissue factor (extrinsic) pathway and the contact factor (intrinsic) pathway. These pathways meet in a final common pathway in which factor Xa converts prothrombin to thrombin, which then cleaves fibrinogen to fibrin. The prothrombin time (PT) is a plasma and test tube test of the integrity of the extrinsic pathway, and the activated clotting time or activated partial thromboplastin time (aPTT) are tests of the intrinsic system for blood and plasma, respectively.
This model based on the concept of a waterfall or cascade is an oversimplification of the coagulation system, as proteins from each pathway influence one another. It is probably more correct to think of the coagulation system as an interactive network with carefully placed amplifiers and restraints.
Fibrin formation involves a process of initiation and amplification. The specific properties of platelets and the coagulation system cooperate to ensure that fibrin formation occurs only at the localized site where it is required to initiate wound repair. This is achieved by the following physicochemical means. First, the surface of resting platelets contains acidic phospholipids such as phosphatidylserine that have their negatively charged pole directed inward. During irreversible shape change, this pole is flipped to the outside of the platelet to provide a negatively charged outer surface to anchor coagulation. Second, the coagulation system relies primarily on soluble factors synthesized in the liver that circulate in the plasma as inactive zymogen forms and become active after proteolytic cleavage. Apart from factor XIII, which is a transglutaminase, all active factors are serine proteases related to the digestive enzyme trypsin. Other factors in the coagulation process, such as tissue factor, factor V, factor VIII, and high-molecular-weight kininogen act as cofactors. Factors VII, IX, X, and prothrombin contain carboxylated glutamic acid residues at their N-terminal regions. Vitamin K acts as a cofactor for the enzyme that carboxylates glutamic acid, forming gamma-carboxy glutamic acid, with a resultant higher density of negative charges. This charged area interacts at the organizing surface of the platelet with ionized calcium ions (Ca 2+ ), acting as a bridge with the negative surface charge on the activated platelet.
Indirect Inhibitors of Thrombin Generation
Historical Considerations
Heparin, one of the oldest anticoagulant drugs currently still in widespread clinical use, was discovered in 1916 by a second-year medical student, Jay McLean, and Professor Howell at Johns Hopkins University. McLean was investigating procoagulant preparations when he isolated a fat-soluble phosphatide anticoagulant in canine liver for which Howell coined the term heparin (from hepar , Greek for liver). In the early 1920s, Howell isolated a water-soluble polysaccharide anticoagulant, which was also termed heparin , although it was distinct from the phosphatide preparations previously isolated.
Research on heparin continued into the 1930s. Jorpes described the structure of heparin in 1935, and the first heparin product for intravenous use was launched in 1936. Best perfected a technique for producing safe, nontoxic heparin that could be administered in a salt solution. The first human trials of heparin began in 1935, and by 1937 it was clear that heparin was a safe, easily available, and effective anticoagulant.
Heparins
Heparins are available as unfractionated heparin (UFH) and low-molecular-weight heparins (LMWHs), which are chemical modifications of unfractionated heparin. The pharmacology of both has been extensively reviewed.
Unfractionated Heparin
UFH is a naturally occurring glycosaminoglycan. It is a negatively charged sulfated polysaccharide formed from alternating residues of D-glucosamine and L-iduronic acid. Heparin is mostly located in lung, intestine, and liver in mammals. Standard preparations are derived from porcine intestine and prepared as calcium or sodium salts. The number and sequence of the saccharides are variable, producing a heterogeneous collection of polysaccharides. Molecular weights range from 3000 to 30,000 Da, with a mean of 15,000 Da representing 40 to 50 saccharides in length. There is no apparent difference between any of the available forms of UFH with respect to pharmacology or anticoagulant profile.
Mechanism of Action
Numerous physiologic actions have been proposed for heparin. Heparin has a direct antiinflammatory action in humans and a wide spectrum of interactions with various enzymes, hormones, biogenic amines, and plasma proteins. The role of heparin as an antiinflammatory agent is strengthened when one considers that heparin alone has no effects on coagulation and is found in lower orders of the animal kingdom, such as mollusks, which lack a coagulation system. Heparin alone has no anticoagulant activity but requires a plasma cofactor, originally designated antithrombin III, and now simply called antithrombin or AT . AT has a low level of intrinsic anticoagulant activity mediated by a positively charged arginine center that binds to the catalytic serine of proteases of the coagulation cascade.
Binding of heparin to AT is highly specific via a pentasaccharide sequence found in about one-third of molecules and is reversible. Binding of heparin and subsequent anticoagulant activity depend on saccharide chain length ( Fig. 45.2 ). The binding of AT to thrombin is suicidal to the thrombin-antithrombin complex, but the heparin molecule is able to dissociate from this complex unchanged. Inhibition of thrombin activation also prevents feedback by thrombin on factors V and VIII, which normally amplifies the clotting cascade. In contrast, inhibition of factors IXa, Xa, and XIIa requires only that heparin bind AT to form the heparin-AT complex. This explains why LMWHs and the synthetic pentasaccharide fondaparinux act as inhibitors of factor Xa but not necessarily of thrombin.

Heparin has actions in addition to anticoagulant effects mediated via AT. At high blood levels (>4 IU/mL) heparin is capable of binding heparin cofactor II, potentiating its inactivation of bound activated thrombin. This action does not require the specific AT binding site but does require heparins of greater than 7200 Da or 24 saccharide units in length. The clinical importance of this action is unclear, but it might account for the need for higher doses of heparin to inhibit clot-bound thrombin. Much higher levels of heparin are needed to prevent the extension of venous thrombosis compared with those required to inhibit initiation of thrombosis. Heparin also stimulates release of tissue factor plasma inhibitor, which binds and neutralizes the tissue factor–FVIIa complex, reducing prothrombinase production via the extrinsic pathway. Plasma concentrations of tissue factor plasma inhibitor (TFPI) rise twofold to sixfold after injection of UFH and LMWH.
Heparin also impairs platelet aggregation mediated by vWF and collagen, and inhibits platelet function by direct binding to platelets. The higher-molecular-weight heparins interfere most with platelet function. These actions might contribute to heparin-induced hemorrhage by a mechanism separate from its anticoagulant actions.
Metabolism and Elimination
Elimination of heparin is nonlinear and occurs by two separate processes. The rapid saturable phase of heparin clearance is thought to be due to cellular degradation. Macrophages internalize heparin, then depolymerize and desulfate it; saturation occurs when all receptors have been used and further clearance depends on new receptor synthesis. This process accounts for the poor bioavailability after low-dose subcutaneous injection, in that the slow rate of absorption barely exceeds the capacity of cellular degradation. Significant plasma levels can only be achieved once these cellular receptors have been saturated after a loading dose. The slower phase of heparin elimination is due to renal excretion. As the dose of heparin is increased, elimination half-life increases and the anticoagulant response is exaggerated. At a dose of 25 U/kg the half-life is about 30 minutes, increasing to about 150 minutes with a bolus dose of 400 U/kg. There are no consistent reports of the effects of renal or hepatic dysfunction on the pharmacokinetics of heparin.
Clinical Pharmacology
Pharmacokinetics and Pharmacodynamics
Heparins are usually administered by subcutaneous or intravenous injection as they are poorly absorbed from the gastrointestinal tract and cause hematomas after intramuscular injection. Safety of the two routes is comparable. Intravenous injection is the preferred route for a rapid anticoagulant effect; however, similar levels of anticoagulation can be achieved by the subcutaneous route with a delayed time to maximum effect.
There is great variability in plasma concentration of heparin in relation to dose. After intravenous injection, more than 50% of heparin circulates bound to plasma proteins, including platelet factor 4, histidine-rich glycoprotein, vitronectin, fibronectin, and vWF. The first three of these neutralize heparin activity and its bioavailability. Increased levels of these proteins might account for heparin resistance sometimes seen in malignancy and inflammatory disorders. Plasma levels also decline rapidly owing to redistribution and uptake by endothelial cells and macrophages.
Therapeutic Effects
Heparin is given to reduce thrombin generation and activity and therefore “anticoagulate” the patient. The therapeutic target dose depends on the indication and is tailored to patient need. For example, the degree of anticoagulation required to prevent thrombosis in a hemodialysis system is not as great as that required in cardiopulmonary bypass. Typically effectiveness of the dose is assessed at regular intervals using a coagulation test initiated by contact activation. The plasma version of this is the aPTT, which measures the effect of heparin on thrombin and factors IXa and Xa. The therapeutic range most commonly quoted is aPTT between 1.5 and 2.5 times the control value. However, commercially available kits for measurement of aPTT differ in their sensitivity to heparins. The whole blood version of the aPTT usually used when higher doses are administered is the automated or activated coagulation time (ACT), a standard of care in cardiac surgical practice; typically the ACT is maintained above 400 to 480 seconds. As there is a marked variation in response between individuals to the anticoagulant effect of a fixed dose of unfractionated heparin, regular monitoring of anticoagulation is routine.
Clinical Use
For thromboembolic prophylaxis, UFH is administered either as “low dose” (5000 U subcutaneously 8 or 12 hourly) or “adjusted dose” (3500 U 8 or 12 hourly adjusted to maintain the aPTT about 3 to 5 seconds above control). For treatment of established thromboembolic disease, full therapeutic doses of heparin are used either by intravenous infusion or subcutaneous injection after an intravenous loading dose. Long-term heparin therapy is only used during pregnancy (unlike warfarin, neither UFH nor LMWH are able to cross the placenta) and for recurrent thromboembolic complications while the patient is taking adequate doses of warfarin.
Low-dose UFH is safe and effective prophylactic treatment for surgical patients at risk for VTE. Low-dose subcutaneous UFH produces a greater than 50% reduction in the incidence of venous thrombosis and fatal or nonfatal PE. Although this is associated with an increased incidence of wound hematoma, there is no increase in major or fatal hemorrhage.
Although relatively low doses of heparin are sufficient to provide thromboprophylaxis, much higher concentrations are needed to prevent thrombus propagation. Recommended regimens for the treatment of DVT with UFH include an intravenous loading dose of 5000 to 10,000 U followed by a continuous infusion of 1300 U/hr, adjusted to maintain the aPTT 1.5 to 2.5 times control. This should be continued until warfarin therapy has prolonged the PT to a therapeutic range for at least 24 hours. This regimen reduces recurrence of venous thrombosis and mortality from PE. The most common reason for failure of treatment is inadequate anticoagulation, particularly within the first 24 hours, which is overcome by the large intravenous loading dose. These treatment protocols have been compared with twice-daily subcutaneous LMWHs without laboratory assessment, which is safer and more effective, with significant and important reductions in recurrence of thrombosis and major hemorrhage.
Adverse Effects
Until 2008, the standard of heparin potency was different between heparins marketed in North America, which used the United States Pharmacopeia (USP) standard, and heparins that used the World Health Organization (WHO)’s international standard or international unit. In 2008, the USP adopted new manufacturing controls for heparin, which included a modification to the reference standard for heparin unit dose. These changes were precipitated by a number of cases of severe hypotension, sometimes leading to death, reported in association with administration of heparin. In March 2008, the U.S. Food and Drug Administration (FDA) identified “oversulfated chondroitin sulfate” as a contaminant in heparin originating from China. This contaminant mimics heparin activity and behaves like UFH in standard USP tests. In vitro and in vivo studies showed that oversulfated chondroitin sulfate directly activates the kinin-kallikrein pathway in human plasma, which can lead to generation of bradykinin, a potent vasodilator. In addition, oversulfated chondroitin sulfate induced generation of C3a and C5a, potent anaphylatoxins derived from complement proteins. Screening of plasma samples from various species indicated that swine and humans are sensitive to the effects of oversulfated chondroitin sulfate in a similar manner. Oversulfated chondroitin sulfate–containing heparin and synthetically derived oversulfated chondroitin sulfate induce hypotension associated with kallikrein activation when administered by intravenous infusion in swine. Adopting the WHO international standard standards and introducing other safeguards allow early detection of this contaminant.
The new standardized heparin now has a different strength that results in about a 10% reduction in “anticoagulant” potency compared with the previous USP standard. The current WHO standard has a potency of 122 IU/mg heparin. The reduced potency should have no clinical effect in that dosing regimens are tailored to individual patient needs.
Hemorrhage
Although hemorrhage is rare in patients using prophylactic doses of either UFH or LMWH, it is a frequent complication of therapeutic heparin therapy. With comparable doses, risks are similar using either continuous intravenous or subcutaneous route of administration. Many patient factors increase the risk of hemorrhage, including length of treatment, presence of cardiac, hepatic or renal dysfunction, aspirin or other antiplatelet drug therapy, and recent surgery, trauma, or invasive procedures. Approximately 30% of patients who sustain anticoagulant-related hemorrhage have previously undiagnosed predisposing lesions, particularly of the gastrointestinal and genitourinary tracts. The incidence of major bleeding in anticoagulated patients is approximately 5%. The estimated daily frequency of fatal, major, and all types of hemorrhage in patients receiving therapeutic anticoagulation is 0.05%, 0.8%, and 2%, respectively, which is approximately twice that expected in the absence of anticoagulation.
Heparin-Induced Thrombocytopenia
Heparin-induced thrombocytopenia (HIT) is a relatively common complication with an incidence of 1.1% and 2.3% of patients receiving therapeutic doses of intravenous porcine and bovine heparin, respectively. This usually involves high doses of UFH, but rare cases have been attributed to low-dose subcutaneous heparin prophylaxis or to flushing lines with heparin. The risk of HIT is less with LMWH than with UFH, perhaps because of less interaction with platelets.
Two distinct clinical syndromes have been described. Type I involves mild thrombocytopenia with a platelet count rarely less than 100 × 10 9 /L that occurs during the first few days of treatment and usually recovers rapidly even if heparin is continued. Patients are normally asymptomatic and no specific treatment is required. The underlying mechanism probably involves the action of heparin as a mild platelet aggregator. Type II HIT is characterized by delayed onset of severe, progressive thrombocytopenia with platelet counts less than 100 × 10 9 /L and often less than 50 × 10 9 /L. The platelet count does not recover unless heparin therapy is stopped and recurs promptly if heparin is restarted. Recovery of the platelet count usually occurs within a week but can occasionally be prolonged.
An immune mechanism has been suggested for type II HIT in which heparin binds to platelet factor 4 to stimulate production of an immunoglobulin G antibody. This antibody binds the heparin-platelet factor 4 complex, which is capable of binding to platelets to produce two separate effects. First, the immune complexes coat platelets and increase their removal from the circulation by the reticuloendothelial system. Second, the immune complexes activate platelets and the coagulation cascade, leading to a hypercoagulable state. Hemorrhage is uncommon and resistance to anticoagulation can occur as the result of heparin-induced release of platelet factor 4. A high index of suspicion is necessary because only immediate withdrawal of heparin reduces mortality and morbidity. A clinical score system (4 T’s—Thrombocytopenia, Timing, Thrombosis, oTher causes) as an aid has been validated in clinical practice ( Table 45.1 ). The most serious complication associated with type II HIT is new thromboembolic events owing to platelet-rich thrombi that continue to form until heparin is withdrawn. Arterial and venous thrombosis can occur either alone or together, and multiple sites are often involved. In patients receiving therapeutic doses of porcine heparin, 0.4% exhibit manifestations of thrombosis, most commonly lower limb thrombosis, thrombotic cerebrovascular events, or acute MI.
4 T’s | 2 Points | 1 Point | 0 Point |
---|---|---|---|
Thrombocytopenia | Platelet count fall >50% and platelet nadir >20 × 10 9 /L | Platelet count fall 30%–50% or platelet nadir 10–19 × 10 9 /L | Platelet count fall <30% or platelet nadir <10 × 10 9 /L |
Timing of platelet count fall | Clear onset between days 5 and 10 or platelet fall 1 day or less (prior heparin exposure within 30 days) | Consistent with days 5–10 fall, but not clear (e.g., missing platelet counts); onset after day 10 or fall 1 day or less (prior heparin exposure 30–100 days ago) | Platelet count fall <4 days without recent exposure |
Thrombosis or other sequelae | New thrombosis (confirmed); skin necrosis; acute systemic reaction postintravenous unfractionated heparin bolus | Progressive or recurrent thrombosis; nonnecrotizing (erythematous) skin lesions; suspected thrombosis (not proven) | None |
Other causes for thrombocytopenia | None apparent | Possible | Definite |
a With a total score of 6 to 8, the probability is high (>80%), intermediate with a score of 4 or 5, and low (<5%) with a score of 0 to 3.
Miscellaneous
Heparin administration can cause anaphylactic reactions, osteoporosis after long-term high-dose therapy, suppression of aldosterone synthesis, delayed transient alopecia, priapism, and rebound hyperlipidemia on withdrawal.
Low-Molecular-Weight Heparin
Basic Pharmacology
Structure Activity
LMWHs are produced from UFH by depolymerization. The resulting marked changes in the properties of LMWHs lead to clinical advantages over UFH. LMWHs have mean molecular weights of 4,000 to 6,500 Da, with a range of 2,000 to 10,000 Da. The production methods used and source of UFH lead to significant variations between different commercial preparations ( Table 45.2 ). LMWHs differ in distribution of their fragment molecular weights, potency (anti-Xa, antithrombin, and anticoagulant activities), and consequently in their biodynamic patterns, recommended doses, and efficacy/safety ratio ( Table 45.3 ).
Preparation | Method of Production | Average Molecular Mass (Da) | 2000–6000 Da (%) |
---|---|---|---|
Nadroparin | Nitrous acid depolymerization and fractionation | 4200 | 85 |
Enoxaparin | Benzoylation followed by alkaline depolymerization | 3900 | 75 |
Dalteparin | Nitrous acid depolymerization and gel filtration | 5700 | 80 |
Tinzaparin | Depolymerization with heparinase | 6000 | 64 |
Certoparin | Depolymerization with isoamylnitrate | 5100 | 63 |
Ardeparin | Peroxidative cleavage | 6000 | |
Reviparin | Nitrous acid depolymerization and chromatographic separation | 4000 |
Preparation | POTENCY | Half-Life (min) | Bioavailability (%) | |
---|---|---|---|---|
Anti-Xa Activity (U/mg) | Anti-Xa to IIa Ratio | |||
Unfractionated heparin | 1 | 30–150 | 10–20 | |
Nadroparin | 95 | 3.6 : 1 | 132–162 | 89 |
Enoxaparin | 105 | 3.8 : 1 | 129–180 | 91 |
Dalteparin | 130 | 2.7 : 1 | 119–139 | 87 |
Tinzaparin | 83 | 1.9 : 1 | 111 | 90 |
Certoparin | 88 | 2.0 : 1 | 240 | 90 |
Ardeparin | 100 | 1.9 : 1 | 200 | 90 |
Reviparin | 130 | 3.6 : 1 | 180 | 90 |
Mechanism of Action
The molecular weight profiles of LMWHs vary significantly, so their anticoagulant properties are not identical. The exact mechanisms underlying the anticoagulant effects of LMWH remain uncertain. The proportion of LMWHs containing the critical pentasaccharide responsible for binding AT is less than in UFH, which results in less inhibition of thrombin via AT in vitro. However, the heparin-AT complex inhibits Xa and this action remains intact in LMWH. Although LMWHs have a weak anti-IIa action, their high bioavailability and long half-life mean that the anti-Xa action is four times greater than for UFH. The potency of LMWHs is further increased by their resistance to inactivation by platelet factor 4 and by lack of protein binding. Like UFH, the main action of LMWHs is to prevent formation of prothrombinase and amplification of the coagulation cascade.
Metabolism and Elimination
LMWHs do not bind to endothelial cells or macrophages, and so are not subject to the rapid uptake that UFH incurs. Similar to UFH, they are partially metabolized by desulfation and depolymerization. The affinity of plasma proteins for LMWHs is much less than for UFH so that only 10% is protein bound. This increased bioavailability ensures a more predictable anticoagulant action. LMWHs have nearly complete bioavailability at all doses when given by subcutaneous injection, compared with 40% for low-dose subcutaneous UFH. Urinary excretion of anti-Xa activity for enoxaparin, dalteparin, and nadroparin, all given at doses for prevention of venous thrombosis, is between 3% and 10% of the injected dose. However, these LMWHs differ in the extent of their nonrenal clearance, resulting in different apparent elimination half-life values and relative apparent bioavailability. When given at thromboprophylactic doses, LMWHs do not significantly cross the placenta. Although clearance of LMWH depends on renal excretion, producing a half-life two to four times as long as for UFH, effects of renal dysfunction are not clinically apparent for creatinine clearance greater than 15 mL/min.
Clinical Pharmacology
LMWHs are as safe and effective as UFH in placebo-controlled and comparative trials. Meta-analysis of these trials suggests low-dose LMWHs are slightly superior to low-dose UFH, particularly in reducing the incidence of PE. LMWHs given as prophylaxis cause an increase in wound hematoma formation but no change in the incidence of hemorrhage. In contrast, a significant reduction in major hemorrhage is seen when LMWHs are used to treat established thrombosis.
Pharmacokinetics
Because LMWHs are mainly administered subcutaneously, compared to UFH they are almost completely absorbed and, in contrast to UFH, exhibit linear pharmacokinetics with proportionality between anti-Xa (and anti-IIa in some cases) plasma activity and dose, and stationary distribution volume and clearance processes when the dosage is increased. Unlike UFH, their distribution volume is close to the blood volume.
Pharmacodynamics
Because of differences between LMWHs, the clinical profile of a given LMWH cannot be extrapolated to another or generalized to all LMWHs. These differences also lead to major problems in providing a reference standard to assess potency. This has a huge impact on licensing generic versions of currently marketed drugs.
Adverse Effects
The principal adverse effect of LMWH is hemorrhage. Unlike UFH there is no antidote such as protamine. Protamine reduces some anticoagulant effects of LMWH but is itself a drug with significant side effects. LMWHs have a high incidence of cross-reaction with the heparin-dependent antibody found in HIT. This can be monitored using a platelet aggregation test. If no cross-reaction occurs, successful anticoagulation can be safely undertaken with LMWH.
Pentasaccharide
Basic Pharmacology
Structure Activity and Mechanism of Action
Fondaparinux is a synthetic preparation of the pentasaccharide sequence found in heparin manufactured to a high degree of purity and uniformity. The antithrombotic activity of fondaparinux is the result of AT-mediated selective inhibition of factor Xa. By selectively binding to AT, fondaparinux potentiates (about 300 times) the innate neutralization of factor Xa by AT. Fondaparinux does not interact with AT to inactivate thrombin and has no known effect on platelet function. At the recommended dose, fondaparinux does not affect fibrinolytic activity or bleeding time.
Metabolism and Elimination
In healthy individuals with normal kidney function up to 75 years of age, about 80% of a single subcutaneous dose is eliminated in urine as unchanged fondaparinux in 72 hours with an elimination half-life of 17 to 21 hours. Since the majority of the administered dose is eliminated unchanged, metabolism of fondaparinux has not been investigated.
Clinical Pharmacology
Pharmacokinetics
Fondaparinux administered by subcutaneous injection is rapidly and completely absorbed (absolute bioavailability is 100%). In healthy adults, fondaparinux is highly (about 94%) and specifically bound to AT, and is thus mainly confined to the blood volume. This is reflected in the apparent volume of distribution of 7 to 11 L. It does not bind significantly to other plasma proteins (including platelet factor 4) or red blood cells.
Pharmacodynamics
Anti-Xa activity is used to define the pharmacology of this agent. As with LMWHs there is a need for a standard against which to calibrate this activity and this standard is unique to fondaparinux. Anti-Xa activity increases with increasing drug concentration, reaching maximum values in 2 to 3 hours.
Therapeutic Effects
Fondaparinux is licensed for VTE prevention in high-risk orthopedic surgery patients and in some countries for general surgical or medical patients. It also is licensed for treatment of acute DVT when administered in conjunction with a vitamin K antagonist. Fondaparinux appears more effective than LMWH for VTE prophylaxis in high-risk orthopedic patients. It is as effective and safe as LMWH for prophylaxis in general surgical patients, and as heparin or LMWH for initial VTE treatment.
Special Populations
Renal Impairment
Fondaparinux elimination is prolonged in patients with renal impairment in that the major route of elimination is urinary excretion of unchanged drug. In patients undergoing prophylaxis after elective hip surgery or hip fracture surgery, the clearance of fondaparinux is 25% lower in patients with mild renal impairment (creatinine clearance [CrCl] 50–80 mL/min), 40% lower in patients with moderate renal impairment (CrCl 30–50 mL/min), and 55% lower in patients with severe renal impairment (CrCl < 30 mL/min) compared with patients with normal renal function.
Hepatic Impairment
In patients with moderate hepatic impairment (Child-Pugh category B), small changes in pharmacokinetics but not pharmacodynamic parameters such as aPTT, PT/international normalized ratio (INR), and AT have been observed. Based on these data, no dosage adjustment is recommended for hepatic impairment. However, a higher incidence of hemorrhage has been noted in subjects with moderate hepatic impairment than in normal subjects.
Age and Frailty
Fondaparinux elimination is prolonged in patients older than 75 years with total clearance 25% lower compared with patients younger than 65 years. Total clearance is also decreased by 30% in patients weighing less than 50 kg. The second-generation pentasaccharides, idraparinux and idrabiotaparinux, which have a longer half-life, have had their development suspended mainly because of an increased risk of bleeding.
Vitamin K Antagonists
Historical Considerations
Following an outbreak of a previously unrecognized hemorrhagic cattle disease in the northern United States and Canada in the 1920s, a Canadian veterinary pathologist determined that the cattle were ingesting moldy sweet clover that functioned as a potent anticoagulant. In 1933, Link, working at the University of Wisconsin, isolated and characterized the hemorrhagic agent as 3,3′-methylene-bis(4-hydroxycoumarin), later named dicoumarol . Over the next few years, numerous similar chemicals were found to have the same anticoagulant properties. The first drug in the class to be widely commercialized was dicoumarol itself, patented in 1941 and later used as a pharmaceutical. Link continued working on developing more potent coumarin-based anticoagulants for use as rodent poisons, resulting in warfarin in 1948. The name warfarin stems from the acronym WARF, for Wisconsin Alumni Research Foundation plus the ending -arin indicating its link with coumarin. Studies on the use of warfarin as a therapeutic anticoagulant found it to be generally superior to dicoumarol, and in 1954 it was approved for medical use in humans. The exact mechanism of action remained unknown until it was demonstrated in 1978 that warfarin inhibits epoxide reductase and hence interferes with vitamin K metabolism.
Basic Pharmacology
Mechanism of Action
Warfarin inhibits vitamin K–dependent synthesis of biologically active forms of clotting factors II, VII, IX, and X, as well as the regulatory factors protein C and protein S. The precursors of these factors require carboxylation of specific glutamic acid residues to allow the coagulation factors to bind to phospholipid surfaces such as that of activated platelets. The enzyme that carries out carboxylation of glutamic acid is gamma-glutamyl carboxylase. The carboxylation reaction proceeds only if this enzyme is able to convert reduced vitamin K (vitamin K hydroquinone) to vitamin K epoxide. Vitamin K epoxide is in turn recycled back to vitamin K and vitamin K hydroquinone by vitamin K epoxide reductase (VKOR; Fig. 45.3 ). Warfarin inhibits epoxide reductase (specifically the VKORC1 subunit), thereby diminishing available vitamin K and vitamin K hydroquinone, which inhibits the carboxylation activity of glutamyl carboxylase. Coagulation factors not carboxylated are incapable of binding to surface phospholipids and are thus biologically inactive.

Metabolism
Warfarin consists of a racemic mixture of two active enantiomers: R and S forms. S-warfarin has five times the potency of the R-isomer with respect to vitamin K antagonism. The enantiomers of warfarin are differentially metabolized by human cytochrome P450 (CYP). R-warfarin is metabolized primarily by CYP 1A2 to 6- and 8-hydroxywarfarin, by CYP 3A4 to 10-hydroxywarfarin, and by carbonyl reductase to diastereoisomeric alcohols. S-warfarin is metabolized primarily by CYP 2C9 to 7-hydroxywarfarin (see Fig. 45.3 ). The efficacy of warfarin is affected primarily when metabolism of S-warfarin is altered. Potential warfarin-drug interactions occur with a very wide range of drugs that are metabolized by these CYP isoforms; a large number of such interactions have been reported ( Table 45.4 ).
Drugs That Change Plasma Concentration of Warfarin | Additive Anticoagulant Effect With No Change in Plasma Concentration | Unknown Mechanisms |
---|---|---|
Prolong Prothrombin Time/Increase Bleeding Risk | ||
|
|
|
|
| |
| ||
Reduce Prothrombin Time/Decrease Bleeding Risk | ||
| Penicillins | |
|
Clinical Pharmacology
Pharmacokinetics
Warfarin has a long half-life (~35 hours) and therefore needs to be given only once daily. It takes several days for warfarin to produce a therapeutic effect because it affects only newly synthesized but not circulating coagulation factors. This also means that it remains effective for several days after administration is stopped. Loading doses of warfarin of more than 5 mg produce a rapid decline in carboxyglutamyl factor VII (half-life 5–7 hours), resulting in an initial prolongation of the INR, but full antithrombotic effect does not occur until a significant reduction in prothrombin activity occurs (half-life about 60 hours) days later. Initiation of warfarin therapy can promote clot formation temporarily because anticoagulant protein C and protein S are also dependent on vitamin K activity. Warfarin causes reduced protein C levels (half-life 14 hours). In addition, reduced levels of protein S (half-life 42 hours) lead to a reduction in activity of protein C (for which it is the cofactor) and therefore reduced inactivation of factor Va and factor VIIIa. The hemostasis system then becomes temporarily biased toward clot formation. Thus it is beneficial to coadminister heparin with initiation of warfarin therapy for 4 to 5 days until the full effect of warfarin has been achieved.
Pharmacodynamics
The effect of warfarin on blood coagulation is measured using an in vitro coagulation test, the PT, or the better standardized INR. Mathematically, INR = (PR) ISI , where PR is the prothrombin ratio (PT divided by the laboratory control PT) and ISI is the international sensitivity index of the thromboplastin reagent. Use of the INR eliminates variation in PT results between laboratories caused by differences in thromboplastin reagents.
There is little correlation between warfarin dose, serum concentration, and therapeutic effect between patients, necessitating individualized dosing guided by therapeutic monitoring of PT or INR. The pharmacokinetic and pharmacodynamic properties of warfarin as well as its narrow therapeutic index make it particularly susceptible to interactions with other drugs ( Table 45.4 ). This necessitates PT monitoring and warfarin dosing adjustments to maintain safe and effective anticoagulation.
Therapeutic Effects
Warfarin is used in a number of chronic thrombotic and thromboembolic conditions. Some of these, together with the recommended INR range for effective therapy or prophylaxis, are listed in Table 45.5 .
Indication | INR |
---|---|
Treatment of venous thrombosis. 6 weeks for calf vein and 3–6 months for proximal deep vein thrombosis | 2.0–3.0 |
Treatment of pulmonary embolism for at least 6 months | 2.0–3.0 |
Prevention of systemic embolism | 2.0–3.0 |
Tissue heart valves | 2.0–3.0 |
To prevent systemic embolism after acute myocardial infarction | 2.0–3.0 |
To prevent recurrent myocardial infarction | 2.5–3.5 |
Valvular heart disease | 2.0–3.0 |
Atrial fibrillation. Older than age 75 years, consider lower range (1.5–2.5) owing to increased risk of intracranial bleed | 2.0–3.0 |
Bileaflet mechanical valve in aortic position | 2.0–3.0 |
Mechanical prosthetic valves with cage | 2.5–3.5 |
Systemic recurrent emboli | 2.5–3.5 |
Adverse Effects
Hemorrhage
The only common side effect of warfarin is hemorrhage. The risk of severe bleeding is small but definite (a median annual rate of 0.9%–2.7% has been reported). Any benefit needs to outweigh this significant risk when warfarin is considered as a therapeutic measure. Risk of bleeding is augmented if the INR is out of range (due to accidental or deliberate overdose, or to drug interactions). This can cause hemoptysis, excessive bruising, bleeding from the nose or gums, or blood in the urine or stool. The risk of bleeding is increased when warfarin is combined with antiplatelet drugs such as clopidogrel, aspirin, or other nonsteroidal antiinflammatory drugs. The risk is also increased in older patients, those prone to falls, and those with trauma or undergoing invasive procedures.
Warfarin Necrosis
A rare but serious complication is warfarin necrosis, which occurs more frequently shortly after commencing treatment in patients with a deficiency of protein C. Protein C requires vitamin K–dependent carboxylation for its activity. Because warfarin initially decreases protein C levels faster than the coagulation factors, it can paradoxically increase coagulation when treatment is initiated, leading to thrombosis typically manifesting as skin necrosis and peripheral gangrene.
Osteoporosis
Several studies have demonstrated a link between warfarin use and osteoporosis-related fracture. A retrospective study of Medicare recipients showed that warfarin use for more than 1 year was linked with a 60% increased risk of osteoporosis-related fracture in men; there was no association in women. The mechanism was thought to be a combination of reduced intake of vitamin K, which is necessary for bone health, and inhibition by warfarin of vitamin K–mediated carboxylation of certain bone proteins, rendering them nonfunctional.
Drug Interactions
Warfarin is the archetypical drug associated with drug interactions and pharmacogenetic effects (see Chapter 4 , Chapter 6 , Chapter 7 ). Hundreds of drugs can increase the risk of hemorrhage in patients taking warfarin, including some nonprescription drugs widely perceived as innocuous. Patients receiving warfarin are typically older and take other medications concomitantly, increasing the chances for drug interactions.
Major interactions that modify bleeding risk with warfarin include the following:
- •
Interference with platelet function: Platelet aggregation is a crucial first step in primary hemostasis (see Chapter 43 ). Drugs that impair platelet function, especially acetylsalicylic acid (aspirin) and clopidogrel, increase the risk of major hemorrhage in patients taking warfarin without elevating the INR. Selective serotonin reuptake inhibitors can inhibit platelet aggregation by depleting platelet serotonin. They also inhibit CYP 2C9, and coadministration with warfarin increases the risk of major bleeding.
- •
Injury to gastrointestinal mucosa: Nonsteroidal antiinflammatory drugs cause dose- and duration-dependent gastrointestinal erosions in a substantial proportion of patients. Most of these erosions are asymptomatic, but the risk of hemorrhage increases with concomitant use of warfarin, even with therapeutic INR.
- •
Altered gut vitamin K availability: Many fruits and vegetables are rich in vitamin K and increase available vitamin K. Most important in this regard are leafy greens such as broccoli, Brussels sprouts, kale, and spinach. The response to warfarin also depends on synthesis of vitamin K 2 (menaquinone) by intestinal microflora. Many antibiotics variably alter the balance of gut flora, thereby enhancing the effect of warfarin.
- •
Interference with warfarin metabolism: Many drugs modify warfarin activity through inhibition or enhancement of CYP metabolic pathways (see Table 45.4 ). Some antibiotics inhibit metabolism of warfarin by CYP enzymes, including cotrimoxazole, metronidazole and, to a lesser extent, the macrolides and fluoroquinolones (see Table 45.4 ).
- •
Interruption of the vitamin K cycle: Some patients experience a rapid and dramatic rise in the INR after standard doses of acetaminophen. Recent evidence suggests that this interaction is caused by N -acetyl (p)-benzoquinoneimine, the highly reactive acetaminophen metabolite that is responsible for hepatic injury after overdose, that also inhibits vitamin K–dependent carboxylase activity.
Pharmacogenetics
Warfarin effect is determined partially by genetic factors. Three single-nucleotide polymorphisms (SNPs), two in the CYP2C9 gene and one in the VKORC1 gene, play key roles in determining the effect of warfarin therapy. The normal variant of the CYP2C9 gene is *1 and the two SNPs *2 and *3. The prevalence of each variant varies by race; 10% and 6% of Caucasians carry the *2 and *3 variants, respectively, but both variants are rare (<2%) in those of African or Asian descent. CYP 2C9*1 metabolizes warfarin normally, CYP2 C9*2 reduces warfarin metabolism by 30%, and CYP 2C9*3 reduces warfarin metabolism by 90%.
In the VKORC1 SNP, the common G allele is replaced by the A allele. Because people with an A allele (or the “A haplotype”) produce less VKORC1 than do those with the G allele (or the “non–A haplotype”), lower warfarin doses are needed to inhibit VKORC1 and to produce an anticoagulant effect. The prevalence of these variants also varies by race, with 37% of Caucasians and 14% of Africans carrying the A allele.
Carriers of CYP 2C9*2 and CYP 2C9*3 require, on average, a 19% and 33% reduction, respectively, per allele in warfarin dose compared with those who carry the *1 allele. Carriers of the VKORC1 A allele require, on average, a 28% reduction in warfarin dose compared with those who carry the G allele. Compared to VKORC1, the CYP 2C9 polymorphisms do not influence time to effective INR but do shorten the time taken to achieve INR greater than 4.
Major drug interactions come from the use of other drugs that use the same CYP pathways for their metabolism or that induce increased enzyme expression, leading to numerous potentially adverse drug interactions. More than 600 drugs reportedly interact with warfarin. The most common and most significant are listed in Table 45.4 .
Special Populations
Pregnancy
Warfarin is contraindicated in pregnancy because it crosses the placenta and can cause bleeding in the fetus. Coumarin (e.g., warfarin) is also teratogenic.
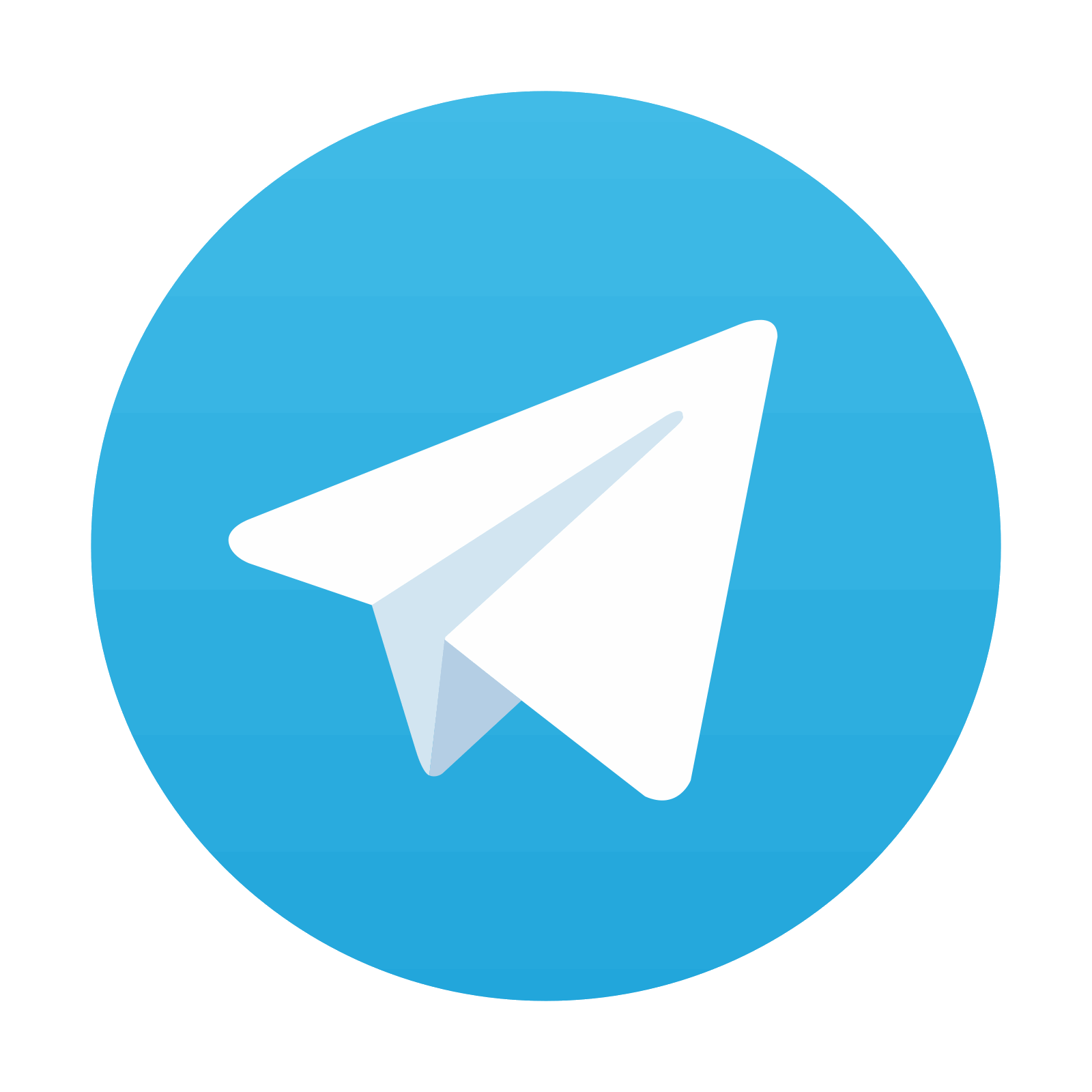
Stay updated, free articles. Join our Telegram channel
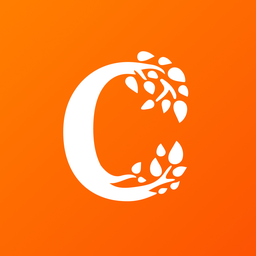
Full access? Get Clinical Tree
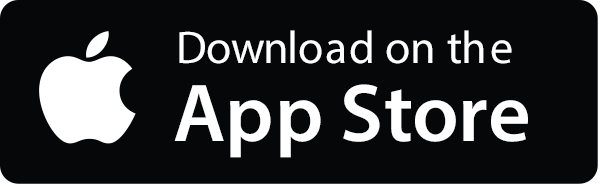
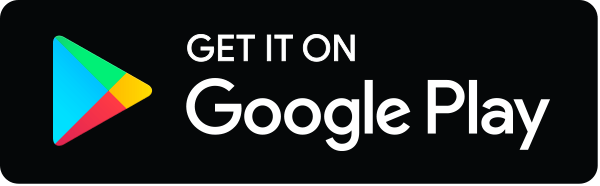